Apr 9, 2025
Apr 9, 2025
Unraveling Degradation: What’s Killing Your Energy Storage?
Unraveling Degradation: What’s Killing Your Energy Storage?

If our last post introduced battery degradation (or aging) as the gradual loss of capacity and performance over time, this one will focus on what drives that degradation at a microscopic level. Battery aging is not caused by a single factor but rather by a combination of degradation mechanisms, each affecting different components of the cell. These mechanisms ultimately lead to a reduction in energy storage capacity, increased resistance, and a decline in overall efficiency.
Understanding these processes is crucial for improving battery technology and extending battery lifespan, which is why battery degradation is an extremely active field of research. While many factors contribute to aging, three of the most widely studied degradation mechanisms are:
Solid Electrolyte Interphase (SEI) Growth: The SEI is a protective layer that forms on the surface of the negative electrode (anode) during battery operation. It acts as a barrier between the electrolyte and the electrode, limiting unwanted side reactions. While this layer is essential for stable battery performance, it doesn’t remain static. Over time, the SEI continues to grow, consuming lithium ions and increasing internal resistance. This leads to capacity fade (less lithium available for charge storage) and reduced efficiency.
Lithium Plating: Under certain conditions—such as fast charging, low temperatures, or high state of charge—metallic lithium can deposit onto the negative electrode surface instead of intercalating properly into the electrode material. This not only reduces available lithium but also increases the risk of dendrite formation. Dendrites are thin, needle-like structures that can pierce the battery separator, leading to internal short circuits and potential safety hazards.
Mechanical Degradation: Every time a battery charges and discharges, its electrode materials expand and contract due to the movement of lithium ions. Over many cycles, this repeated stress can cause cracking of electrode particles, loss of electrical contact, and structural damage to the electrode layers. This mechanical wear accelerates performance loss and makes the battery less efficient over time.
These mechanisms contribute to two primary degradation modes, which define how a battery loses its capacity:
Loss of Lithium Inventory (LLI): When lithium is consumed by unwanted side reactions, such as SEI formation or lithium plating, it is no longer available to participate in the battery’s charge-storage process. Even if the electrodes remain structurally intact, the total usable lithium gradually decreases, reducing the battery’s energy capacity.
Loss of Active Material (LAM): Mechanisms such as electrode particle cracking can lead to a reduction in the amount of material available for charge storage. Unlike LLI, where lithium is lost, LAM affects the electrodes themselves, making them less effective at holding charge. This results not only in lower energy capacity but also in reduced power output, since fewer active sites are available for lithium intercalation.
Both LLI and LAM occur simultaneously, and their relative impact depends on battery chemistry, usage patterns, and environmental conditions. Some degradation mechanisms are dominant in high-temperature environments, while others are more problematic in fast-charging scenarios.
Understanding these degradation mechanisms is key to developing longer-lasting batteries and improving battery health monitoring systems. Over the next few posts, we will take a closer look at each of the major degradation mechanisms, starting with SEI growth.
If our last post introduced battery degradation (or aging) as the gradual loss of capacity and performance over time, this one will focus on what drives that degradation at a microscopic level. Battery aging is not caused by a single factor but rather by a combination of degradation mechanisms, each affecting different components of the cell. These mechanisms ultimately lead to a reduction in energy storage capacity, increased resistance, and a decline in overall efficiency.
Understanding these processes is crucial for improving battery technology and extending battery lifespan, which is why battery degradation is an extremely active field of research. While many factors contribute to aging, three of the most widely studied degradation mechanisms are:
Solid Electrolyte Interphase (SEI) Growth: The SEI is a protective layer that forms on the surface of the negative electrode (anode) during battery operation. It acts as a barrier between the electrolyte and the electrode, limiting unwanted side reactions. While this layer is essential for stable battery performance, it doesn’t remain static. Over time, the SEI continues to grow, consuming lithium ions and increasing internal resistance. This leads to capacity fade (less lithium available for charge storage) and reduced efficiency.
Lithium Plating: Under certain conditions—such as fast charging, low temperatures, or high state of charge—metallic lithium can deposit onto the negative electrode surface instead of intercalating properly into the electrode material. This not only reduces available lithium but also increases the risk of dendrite formation. Dendrites are thin, needle-like structures that can pierce the battery separator, leading to internal short circuits and potential safety hazards.
Mechanical Degradation: Every time a battery charges and discharges, its electrode materials expand and contract due to the movement of lithium ions. Over many cycles, this repeated stress can cause cracking of electrode particles, loss of electrical contact, and structural damage to the electrode layers. This mechanical wear accelerates performance loss and makes the battery less efficient over time.
These mechanisms contribute to two primary degradation modes, which define how a battery loses its capacity:
Loss of Lithium Inventory (LLI): When lithium is consumed by unwanted side reactions, such as SEI formation or lithium plating, it is no longer available to participate in the battery’s charge-storage process. Even if the electrodes remain structurally intact, the total usable lithium gradually decreases, reducing the battery’s energy capacity.
Loss of Active Material (LAM): Mechanisms such as electrode particle cracking can lead to a reduction in the amount of material available for charge storage. Unlike LLI, where lithium is lost, LAM affects the electrodes themselves, making them less effective at holding charge. This results not only in lower energy capacity but also in reduced power output, since fewer active sites are available for lithium intercalation.
Both LLI and LAM occur simultaneously, and their relative impact depends on battery chemistry, usage patterns, and environmental conditions. Some degradation mechanisms are dominant in high-temperature environments, while others are more problematic in fast-charging scenarios.
Understanding these degradation mechanisms is key to developing longer-lasting batteries and improving battery health monitoring systems. Over the next few posts, we will take a closer look at each of the major degradation mechanisms, starting with SEI growth.
If our last post introduced battery degradation (or aging) as the gradual loss of capacity and performance over time, this one will focus on what drives that degradation at a microscopic level. Battery aging is not caused by a single factor but rather by a combination of degradation mechanisms, each affecting different components of the cell. These mechanisms ultimately lead to a reduction in energy storage capacity, increased resistance, and a decline in overall efficiency.
Understanding these processes is crucial for improving battery technology and extending battery lifespan, which is why battery degradation is an extremely active field of research. While many factors contribute to aging, three of the most widely studied degradation mechanisms are:
Solid Electrolyte Interphase (SEI) Growth: The SEI is a protective layer that forms on the surface of the negative electrode (anode) during battery operation. It acts as a barrier between the electrolyte and the electrode, limiting unwanted side reactions. While this layer is essential for stable battery performance, it doesn’t remain static. Over time, the SEI continues to grow, consuming lithium ions and increasing internal resistance. This leads to capacity fade (less lithium available for charge storage) and reduced efficiency.
Lithium Plating: Under certain conditions—such as fast charging, low temperatures, or high state of charge—metallic lithium can deposit onto the negative electrode surface instead of intercalating properly into the electrode material. This not only reduces available lithium but also increases the risk of dendrite formation. Dendrites are thin, needle-like structures that can pierce the battery separator, leading to internal short circuits and potential safety hazards.
Mechanical Degradation: Every time a battery charges and discharges, its electrode materials expand and contract due to the movement of lithium ions. Over many cycles, this repeated stress can cause cracking of electrode particles, loss of electrical contact, and structural damage to the electrode layers. This mechanical wear accelerates performance loss and makes the battery less efficient over time.
These mechanisms contribute to two primary degradation modes, which define how a battery loses its capacity:
Loss of Lithium Inventory (LLI): When lithium is consumed by unwanted side reactions, such as SEI formation or lithium plating, it is no longer available to participate in the battery’s charge-storage process. Even if the electrodes remain structurally intact, the total usable lithium gradually decreases, reducing the battery’s energy capacity.
Loss of Active Material (LAM): Mechanisms such as electrode particle cracking can lead to a reduction in the amount of material available for charge storage. Unlike LLI, where lithium is lost, LAM affects the electrodes themselves, making them less effective at holding charge. This results not only in lower energy capacity but also in reduced power output, since fewer active sites are available for lithium intercalation.
Both LLI and LAM occur simultaneously, and their relative impact depends on battery chemistry, usage patterns, and environmental conditions. Some degradation mechanisms are dominant in high-temperature environments, while others are more problematic in fast-charging scenarios.
Understanding these degradation mechanisms is key to developing longer-lasting batteries and improving battery health monitoring systems. Over the next few posts, we will take a closer look at each of the major degradation mechanisms, starting with SEI growth.
If our last post introduced battery degradation (or aging) as the gradual loss of capacity and performance over time, this one will focus on what drives that degradation at a microscopic level. Battery aging is not caused by a single factor but rather by a combination of degradation mechanisms, each affecting different components of the cell. These mechanisms ultimately lead to a reduction in energy storage capacity, increased resistance, and a decline in overall efficiency.
Understanding these processes is crucial for improving battery technology and extending battery lifespan, which is why battery degradation is an extremely active field of research. While many factors contribute to aging, three of the most widely studied degradation mechanisms are:
Solid Electrolyte Interphase (SEI) Growth: The SEI is a protective layer that forms on the surface of the negative electrode (anode) during battery operation. It acts as a barrier between the electrolyte and the electrode, limiting unwanted side reactions. While this layer is essential for stable battery performance, it doesn’t remain static. Over time, the SEI continues to grow, consuming lithium ions and increasing internal resistance. This leads to capacity fade (less lithium available for charge storage) and reduced efficiency.
Lithium Plating: Under certain conditions—such as fast charging, low temperatures, or high state of charge—metallic lithium can deposit onto the negative electrode surface instead of intercalating properly into the electrode material. This not only reduces available lithium but also increases the risk of dendrite formation. Dendrites are thin, needle-like structures that can pierce the battery separator, leading to internal short circuits and potential safety hazards.
Mechanical Degradation: Every time a battery charges and discharges, its electrode materials expand and contract due to the movement of lithium ions. Over many cycles, this repeated stress can cause cracking of electrode particles, loss of electrical contact, and structural damage to the electrode layers. This mechanical wear accelerates performance loss and makes the battery less efficient over time.
These mechanisms contribute to two primary degradation modes, which define how a battery loses its capacity:
Loss of Lithium Inventory (LLI): When lithium is consumed by unwanted side reactions, such as SEI formation or lithium plating, it is no longer available to participate in the battery’s charge-storage process. Even if the electrodes remain structurally intact, the total usable lithium gradually decreases, reducing the battery’s energy capacity.
Loss of Active Material (LAM): Mechanisms such as electrode particle cracking can lead to a reduction in the amount of material available for charge storage. Unlike LLI, where lithium is lost, LAM affects the electrodes themselves, making them less effective at holding charge. This results not only in lower energy capacity but also in reduced power output, since fewer active sites are available for lithium intercalation.
Both LLI and LAM occur simultaneously, and their relative impact depends on battery chemistry, usage patterns, and environmental conditions. Some degradation mechanisms are dominant in high-temperature environments, while others are more problematic in fast-charging scenarios.
Understanding these degradation mechanisms is key to developing longer-lasting batteries and improving battery health monitoring systems. Over the next few posts, we will take a closer look at each of the major degradation mechanisms, starting with SEI growth.
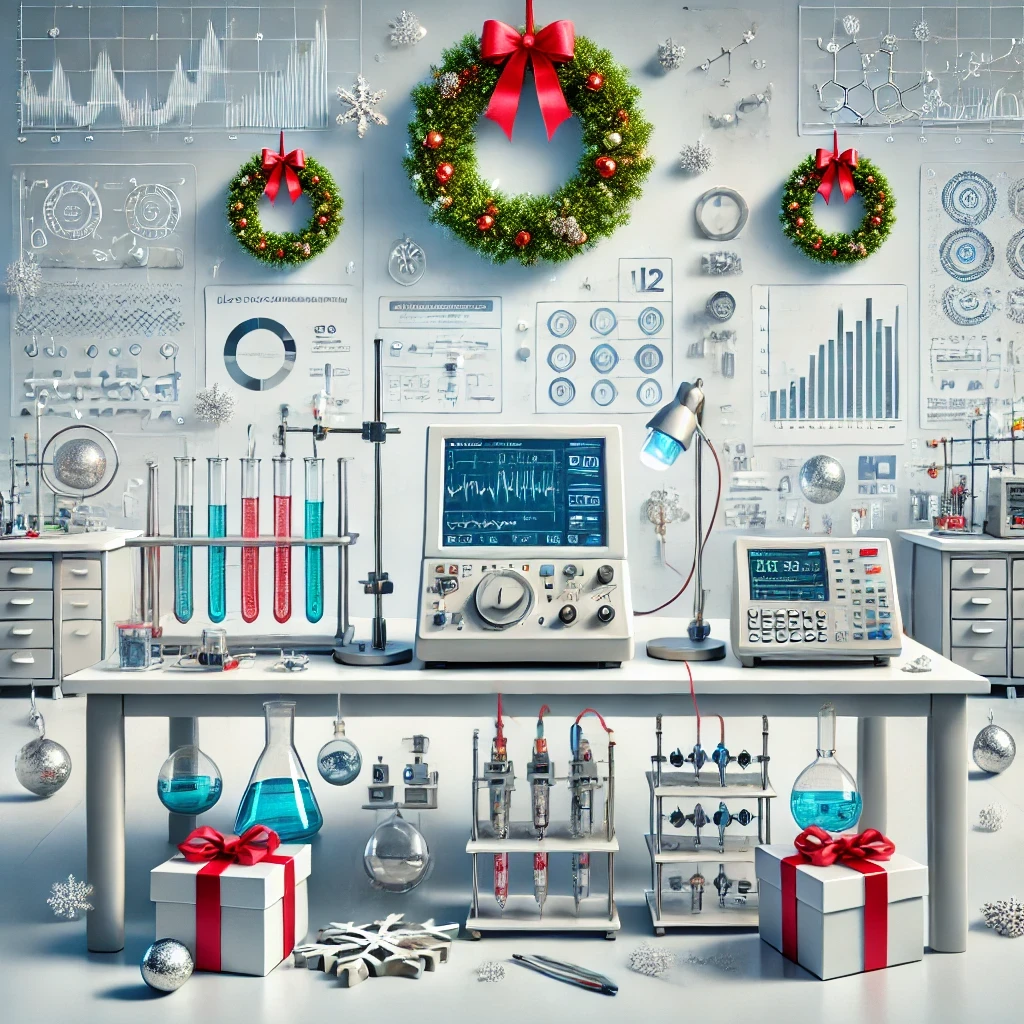
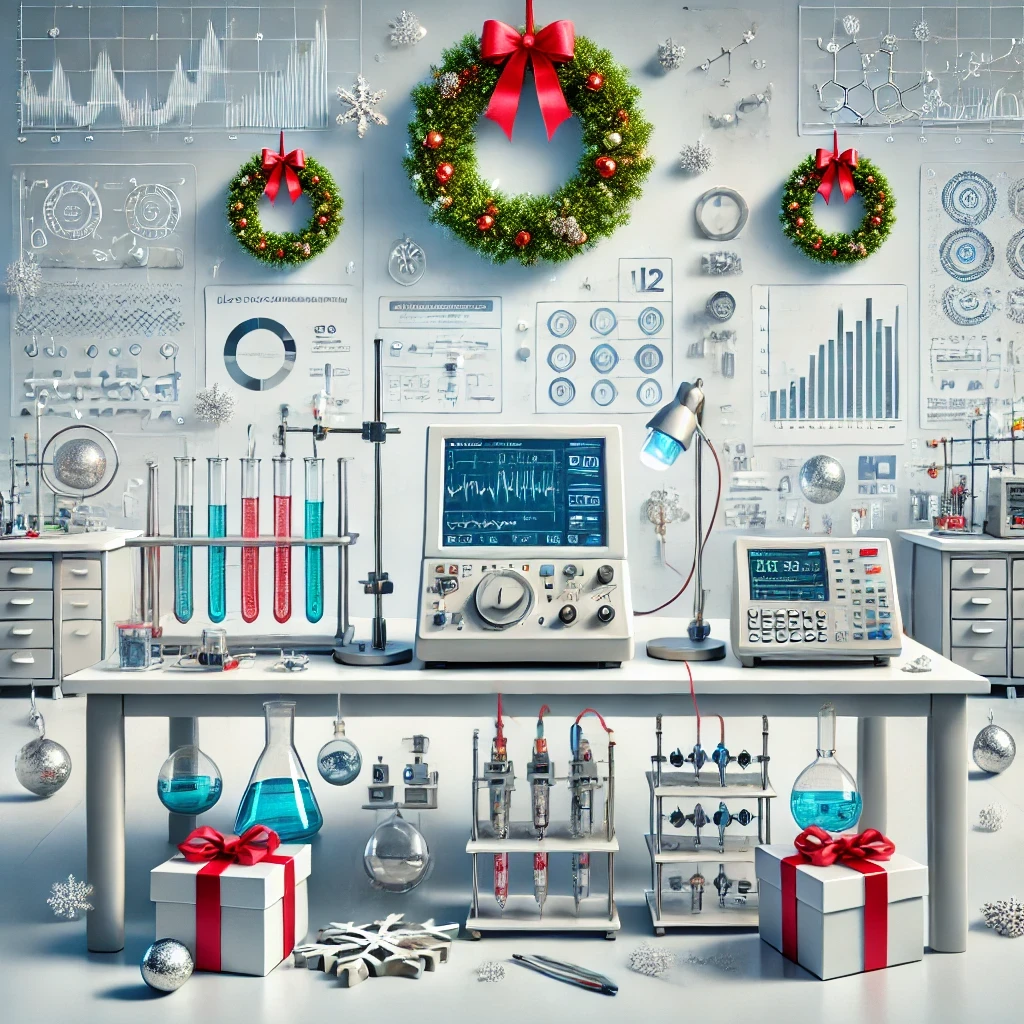
12 Days of Electrochemical Testing
To celebrate the holiday season and the re-release of Ionworks Studio, we featured "12 (business) days of electrochemical testing". Each day we pick a test, give a little bit of information about it, and show you how to run it in Ionworks. 🔋 🎄
Dec 17, 2024
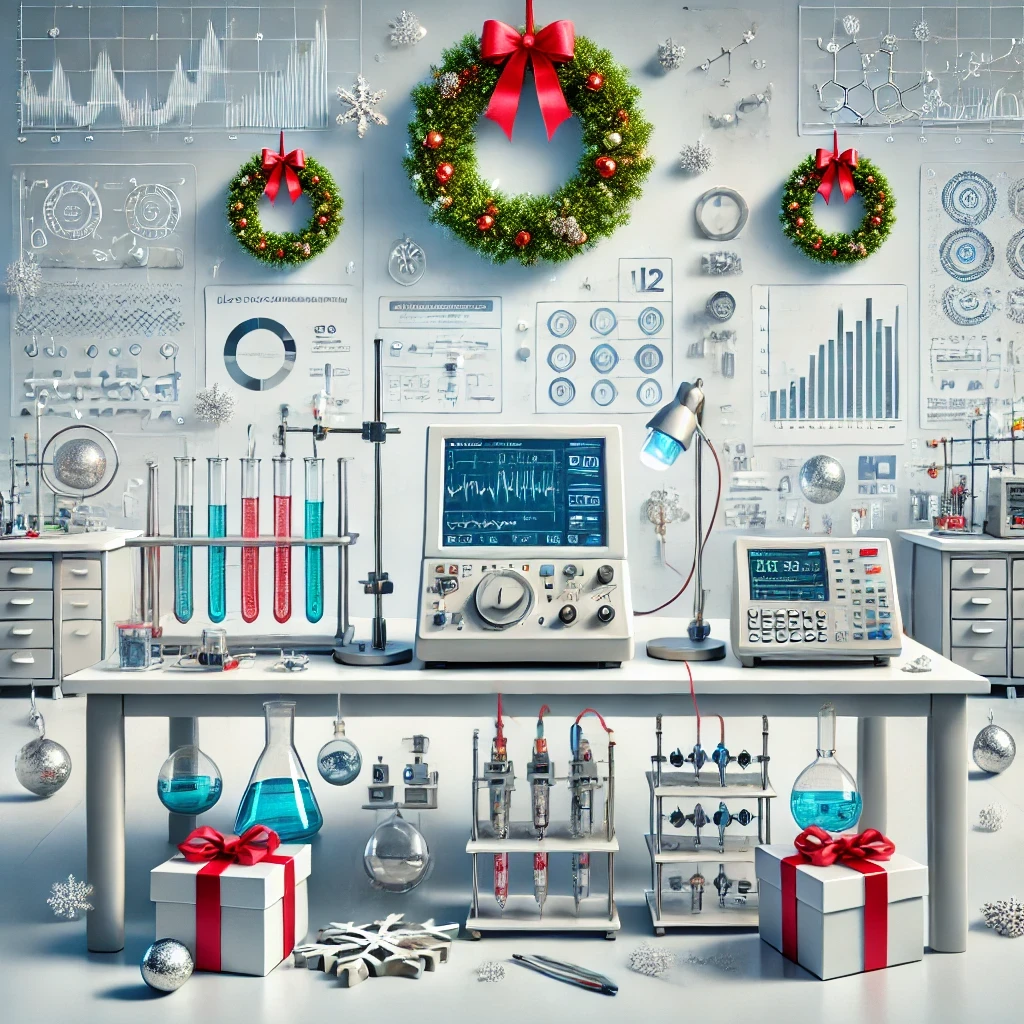
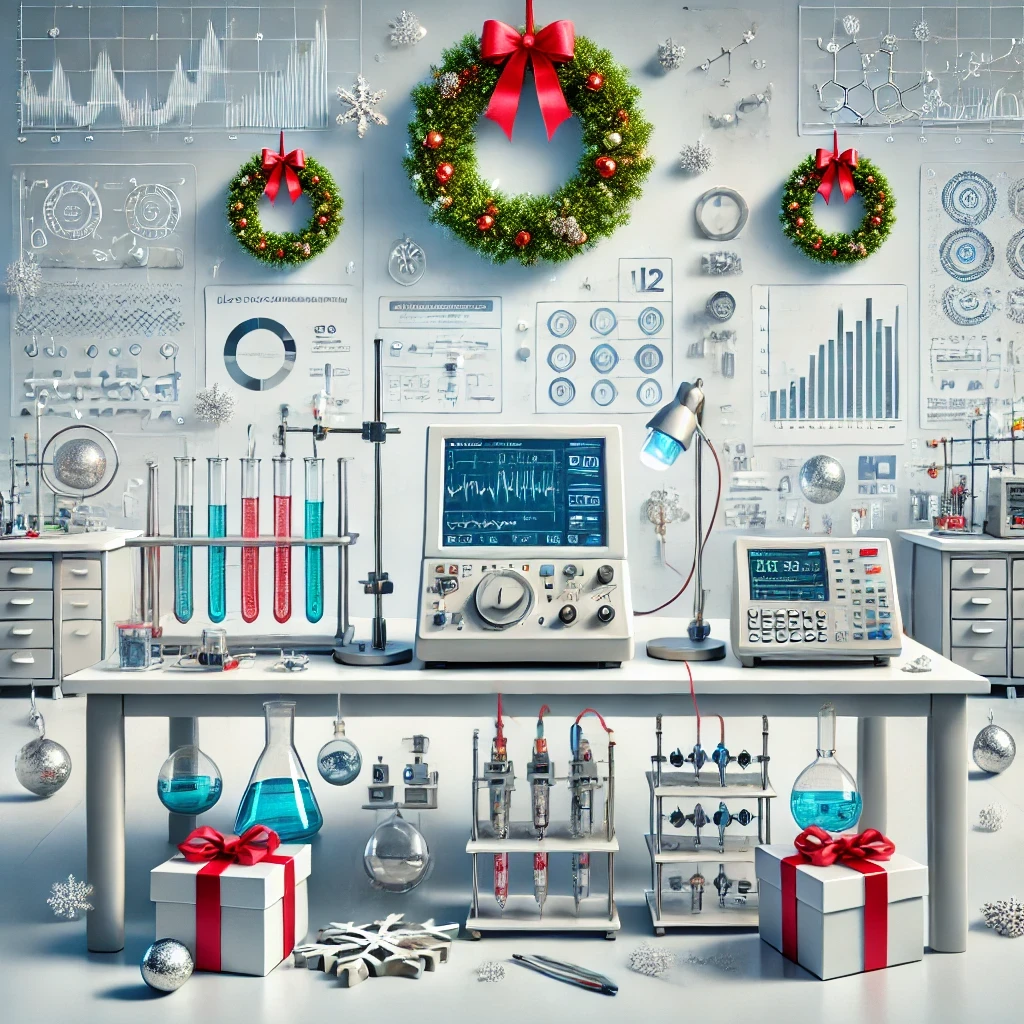
12 Days of Electrochemical Testing
To celebrate the holiday season and the re-release of Ionworks Studio, we featured "12 (business) days of electrochemical testing". Each day we pick a test, give a little bit of information about it, and show you how to run it in Ionworks. 🔋 🎄
Dec 17, 2024
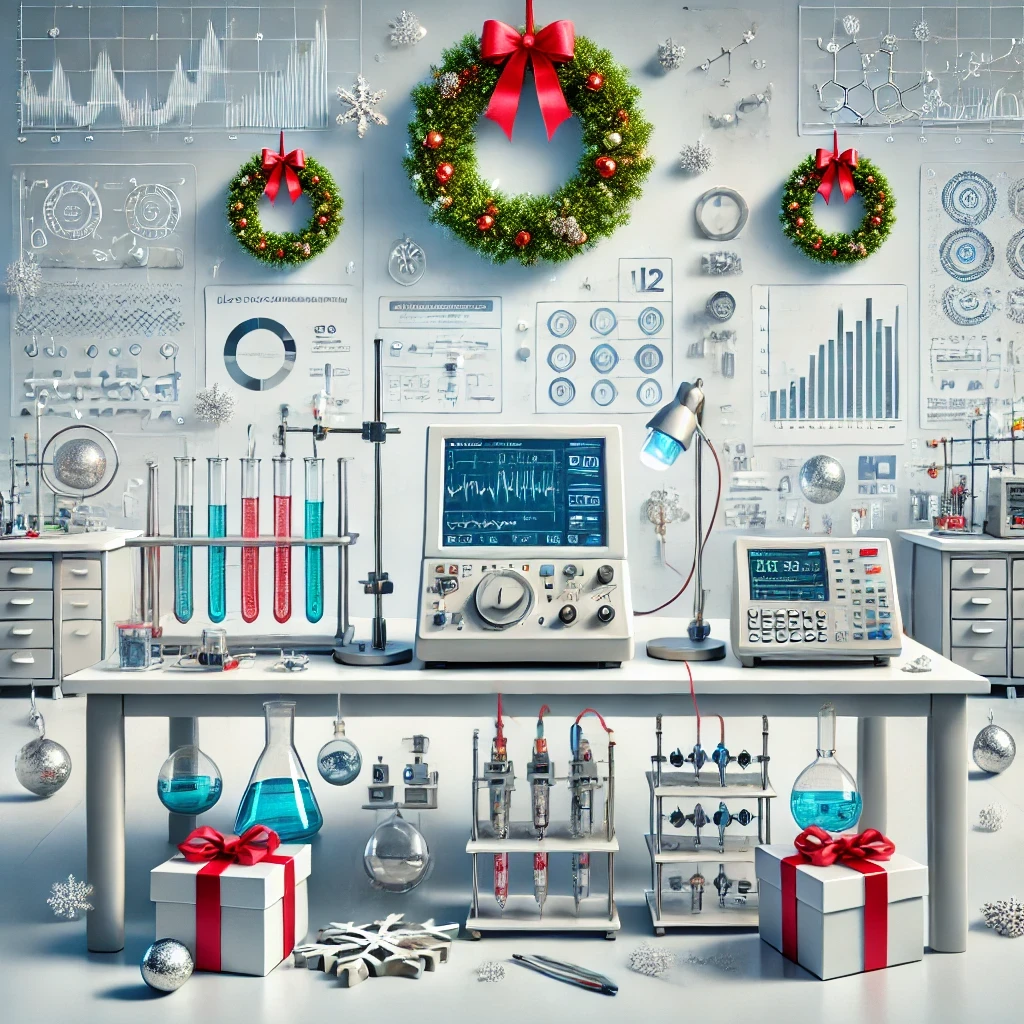
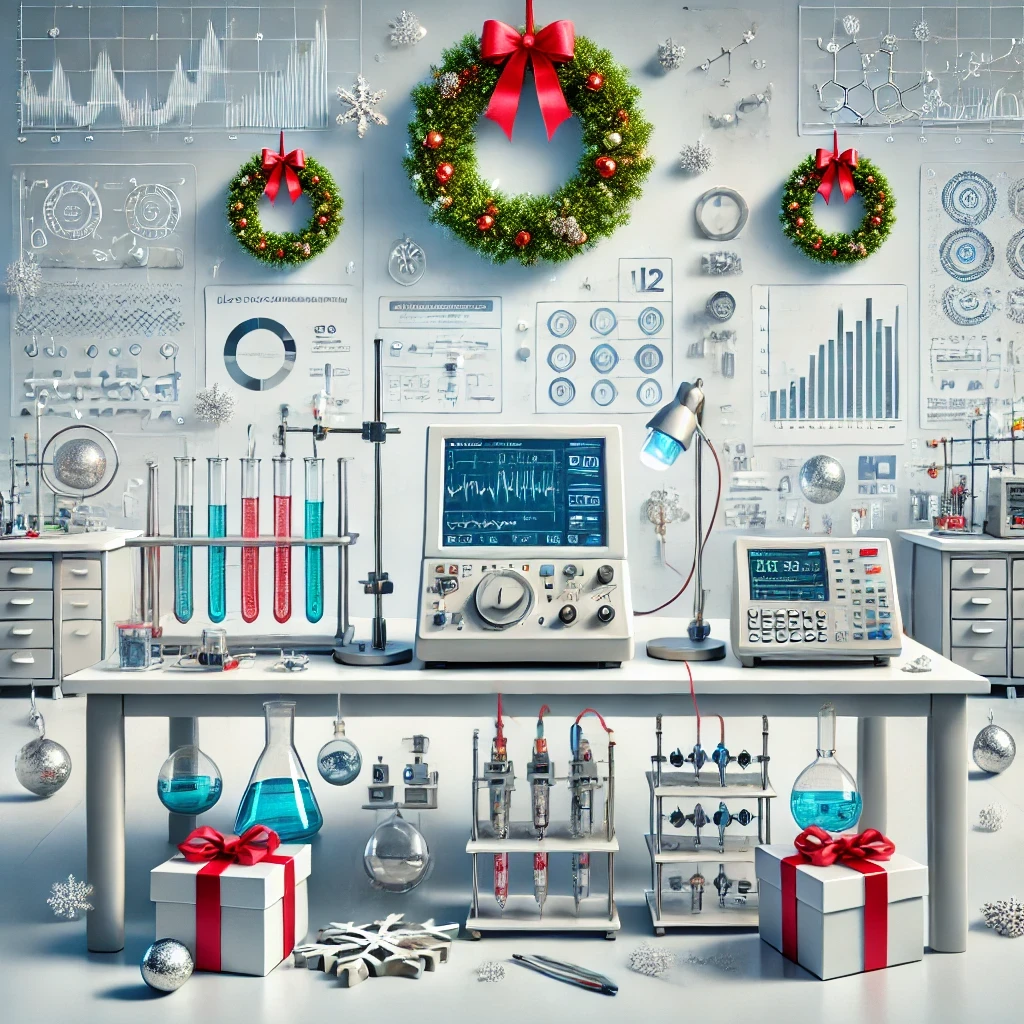
12 Days of Electrochemical Testing
To celebrate the holiday season and the re-release of Ionworks Studio, we featured "12 (business) days of electrochemical testing". Each day we pick a test, give a little bit of information about it, and show you how to run it in Ionworks. 🔋 🎄
Dec 17, 2024
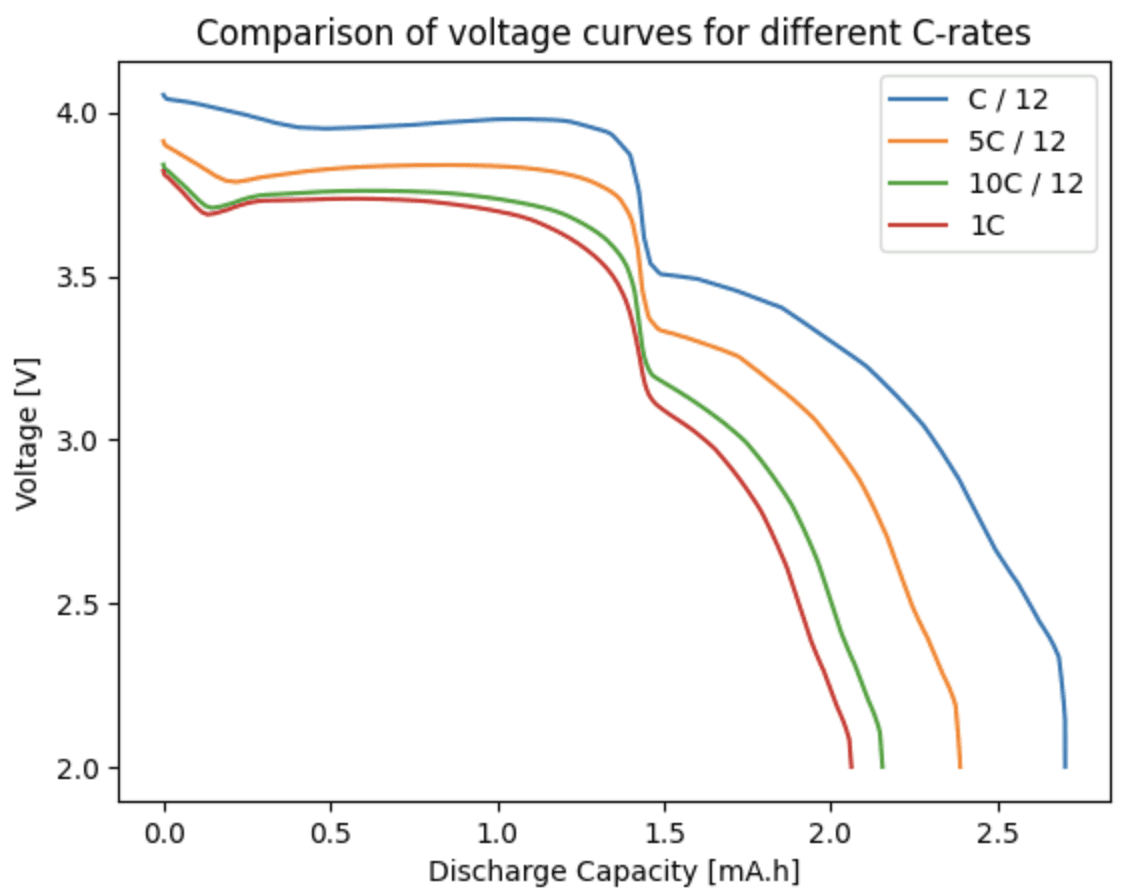
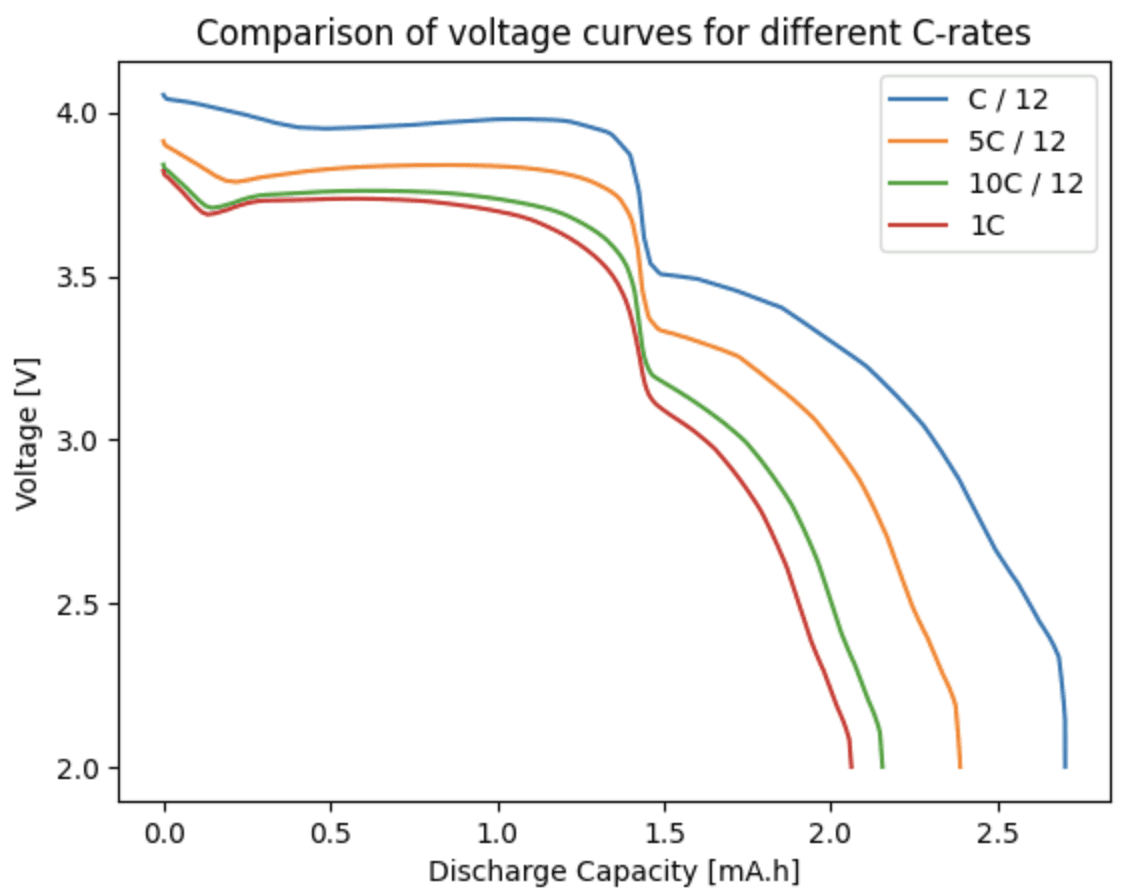
Sodium Ion Battery Model now available in PyBaMM!
This blog post explores the history of SIBs, the intricacies of the new PyBaMM model, and the exciting possibilities it unlocks for the future of energy storage.
Nov 12, 2024
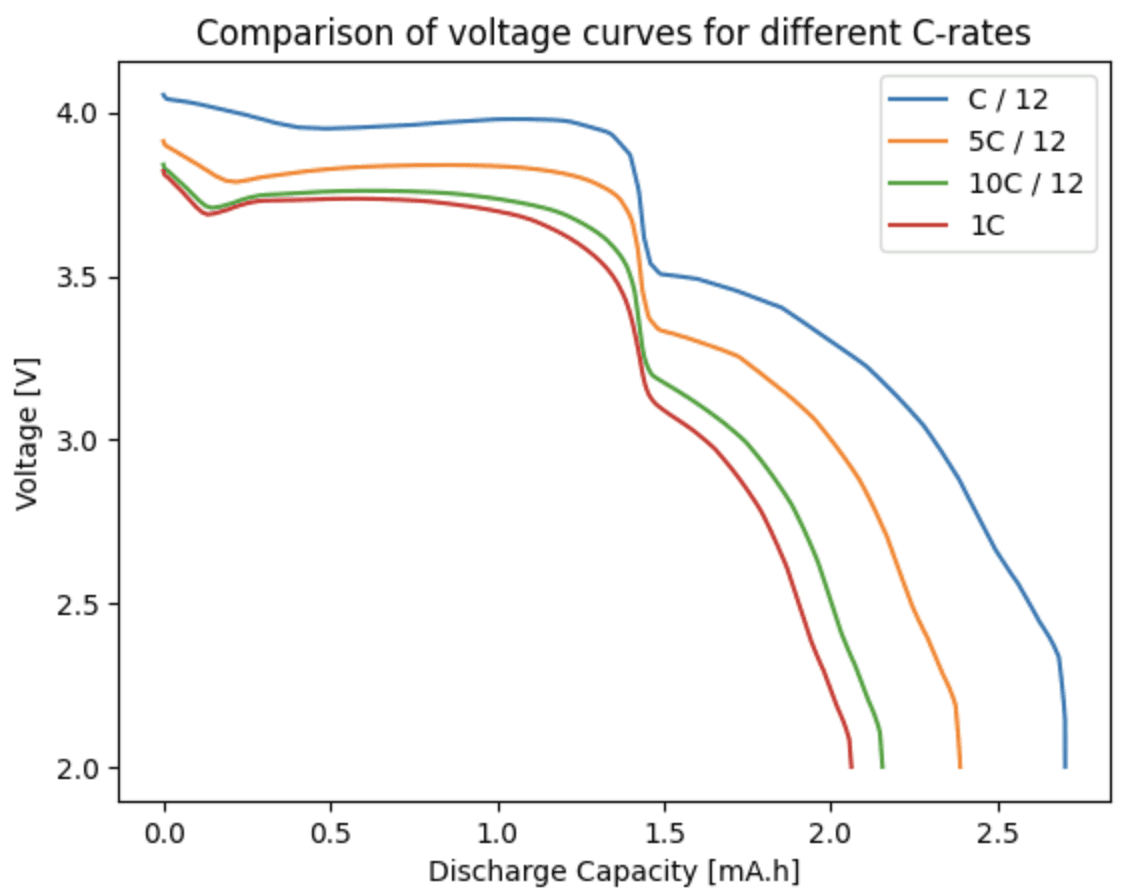
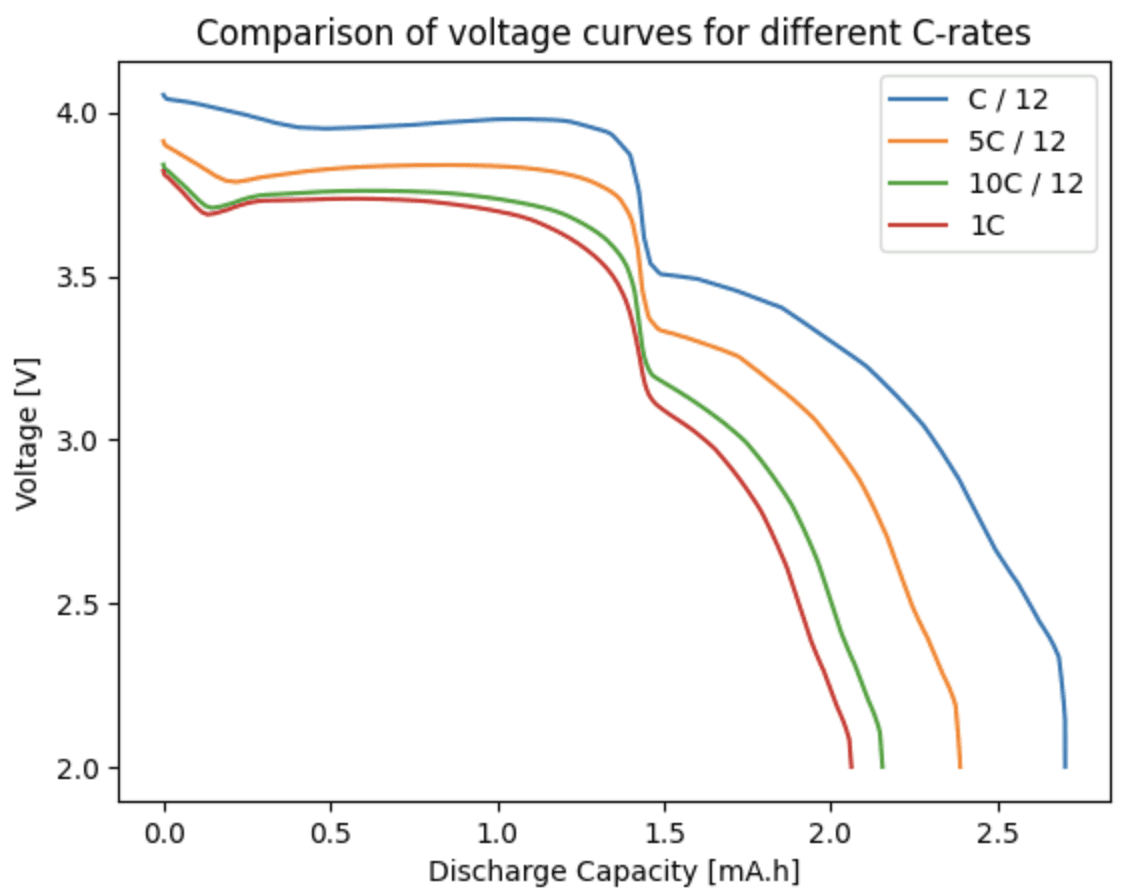
Sodium Ion Battery Model now available in PyBaMM!
This blog post explores the history of SIBs, the intricacies of the new PyBaMM model, and the exciting possibilities it unlocks for the future of energy storage.
Nov 12, 2024
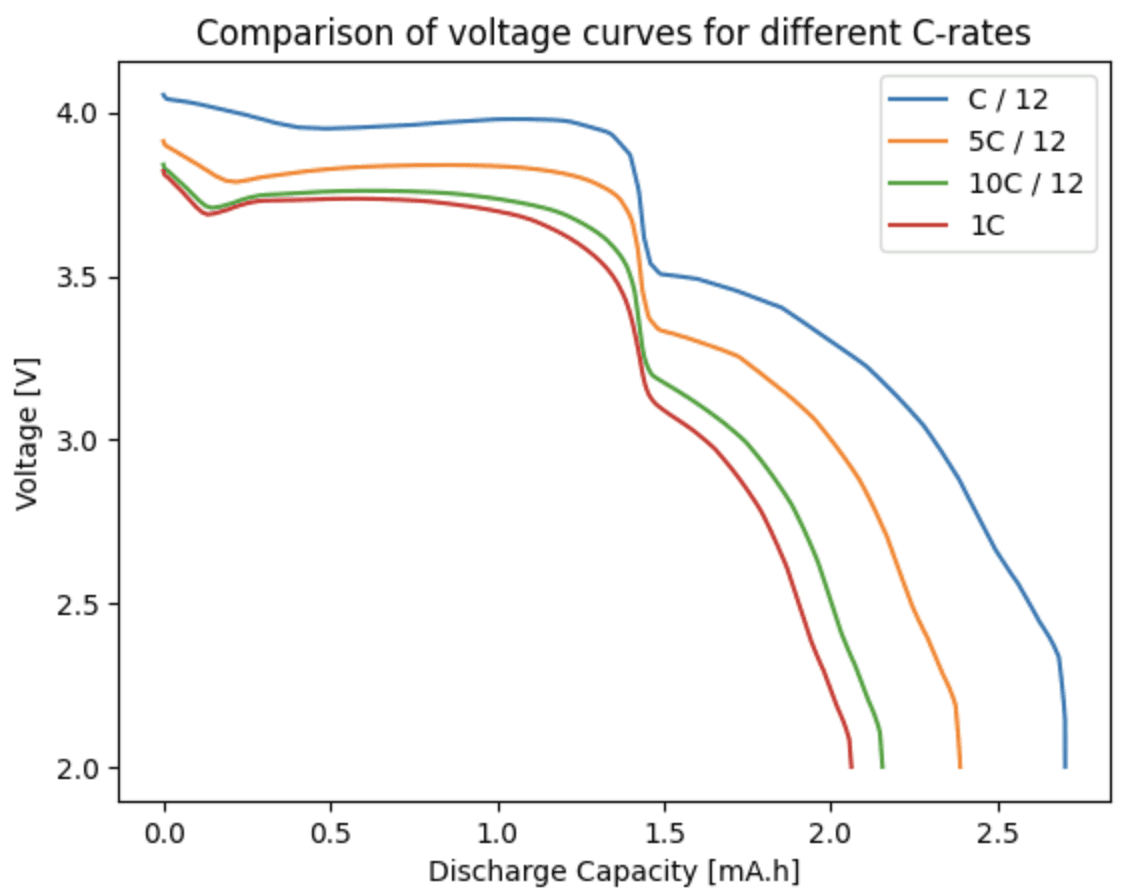
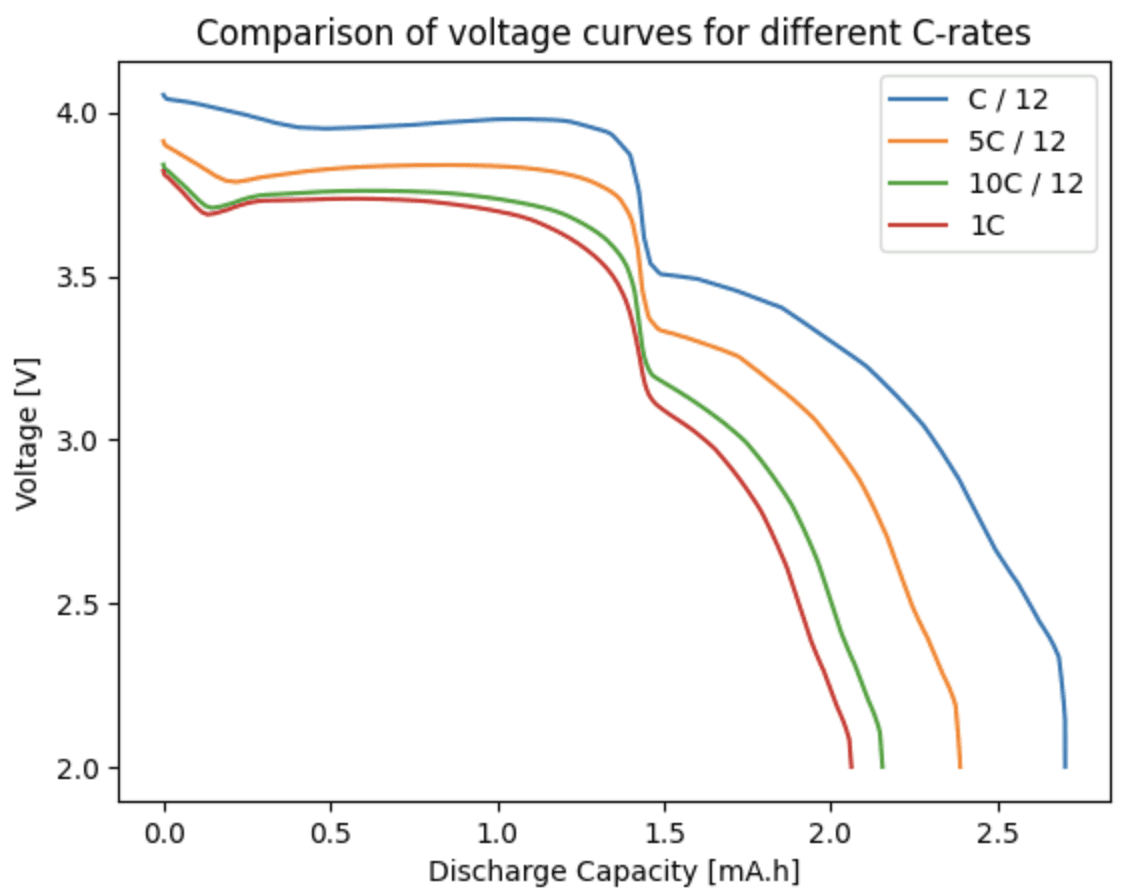
Sodium Ion Battery Model now available in PyBaMM!
This blog post explores the history of SIBs, the intricacies of the new PyBaMM model, and the exciting possibilities it unlocks for the future of energy storage.
Nov 12, 2024
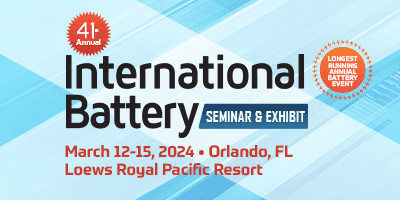
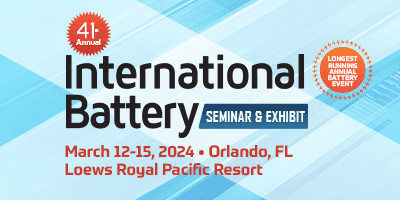
Ionworks Presents at International Battery Seminar
Ionworks CEO Valentin Sulzer presents at the International Battery Seminar in Florida
Mar 12, 2024
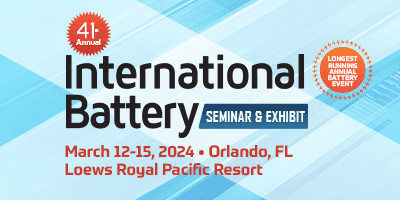
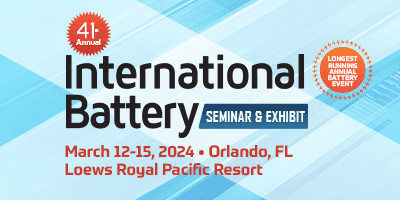
Ionworks Presents at International Battery Seminar
Ionworks CEO Valentin Sulzer presents at the International Battery Seminar in Florida
Mar 12, 2024
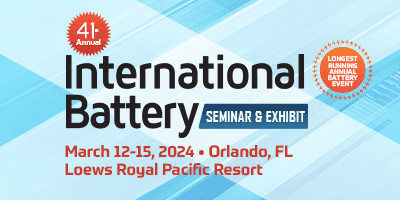
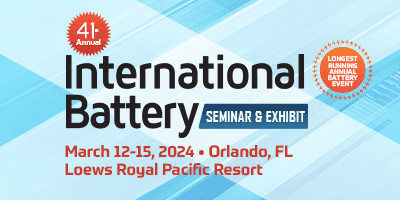
Ionworks Presents at International Battery Seminar
Ionworks CEO Valentin Sulzer presents at the International Battery Seminar in Florida
Mar 12, 2024
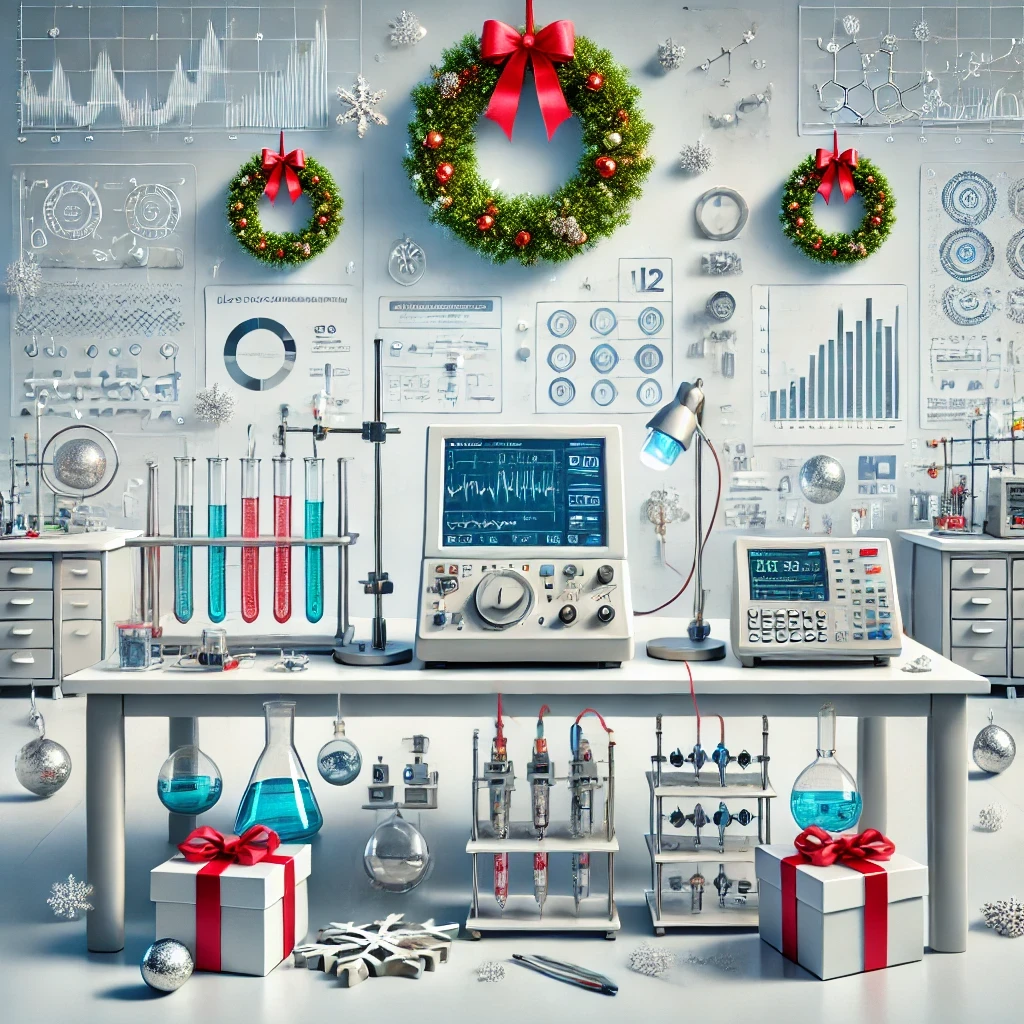
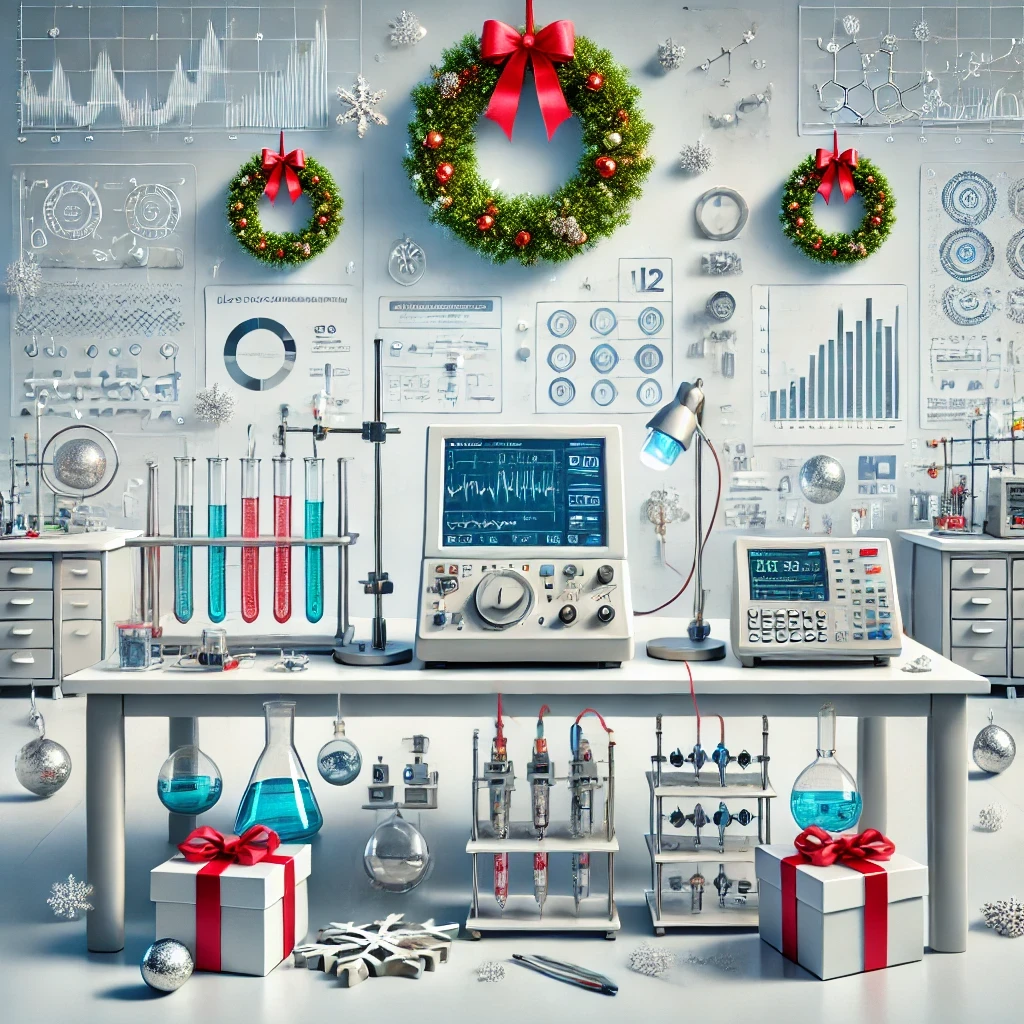
12 Days of Electrochemical Testing
To celebrate the holiday season and the re-release of Ionworks Studio, we featured "12 (business) days of electrochemical testing". Each day we pick a test, give a little bit of information about it, and show you how to run it in Ionworks. 🔋 🎄
Dec 17, 2024
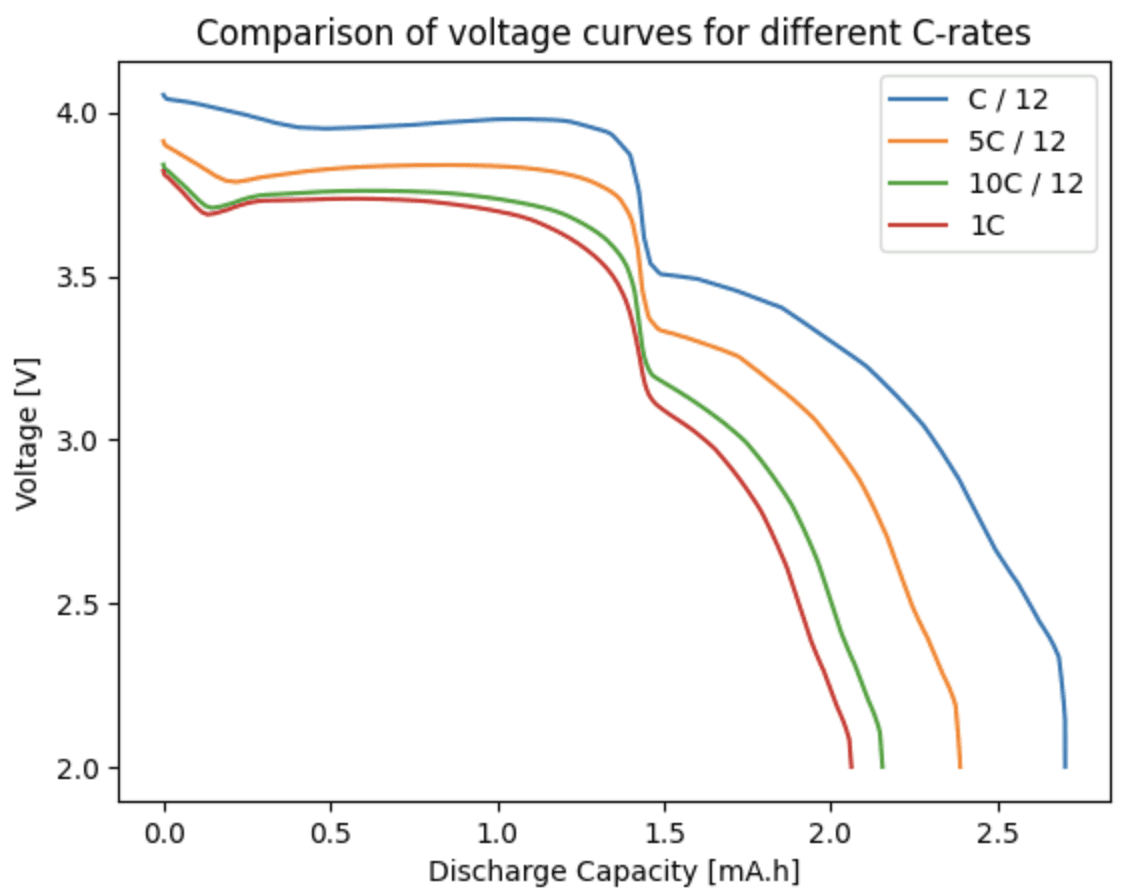
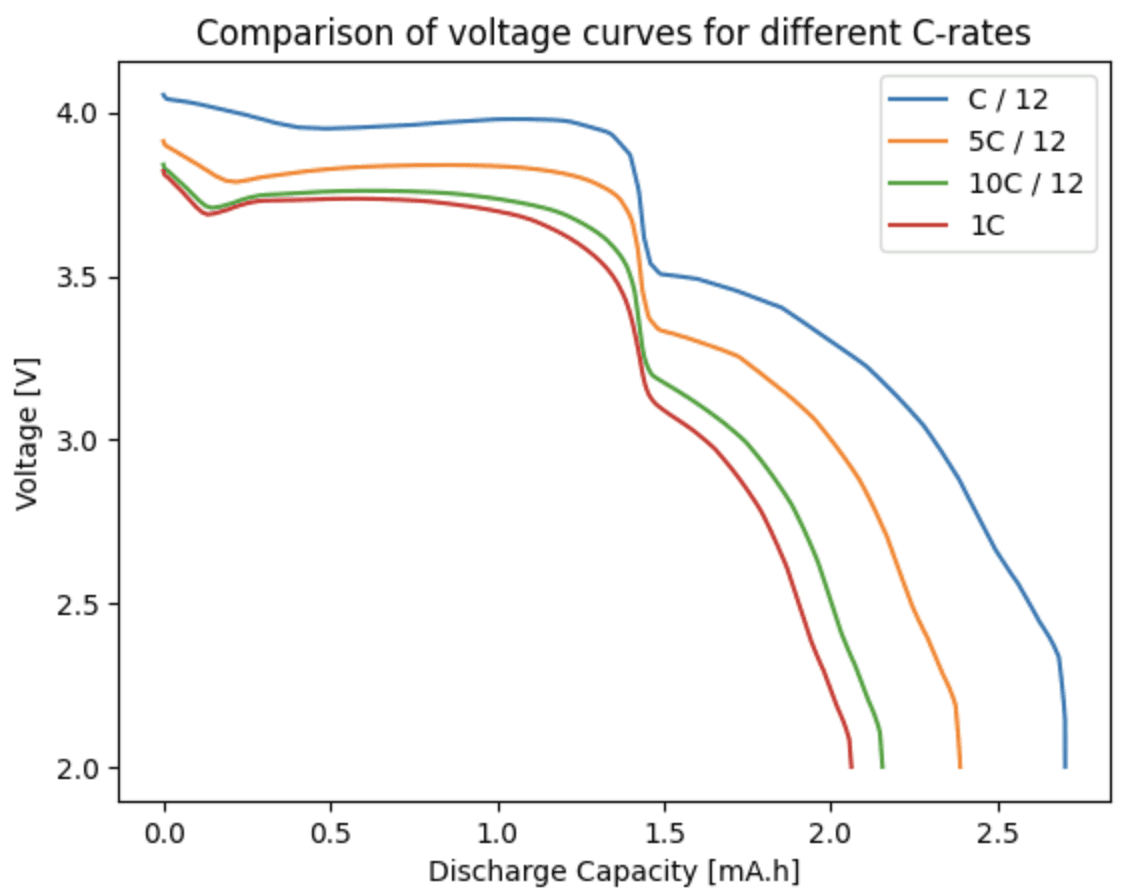
Sodium Ion Battery Model now available in PyBaMM!
This blog post explores the history of SIBs, the intricacies of the new PyBaMM model, and the exciting possibilities it unlocks for the future of energy storage.
Nov 12, 2024
Run your first virtual battery test today
Simulate, iterate, and validate your cell configurations with no lab time required.
Ionworks Technologies Inc. All rights reserved.
Run your first virtual battery test today
Simulate, iterate, and validate your cell configurations with no lab time required.
Run your first virtual battery test today
Simulate, iterate, and validate your cell configurations with no lab time required.
Ionworks Technologies Inc. All rights reserved.
Run your first virtual battery test today
Simulate, iterate, and validate your cell configurations with no lab time required.
Ionworks Technologies Inc. All rights reserved.