Apr 2, 2025
Apr 2, 2025
The Key to Voltage: Understanding the Open-Circuit Voltage
The Key to Voltage: Understanding the Open-Circuit Voltage

We discussed in our previous post how the electrode with a higher potential becomes the positive electrode in a battery. But what exactly do we mean by "potential"? And why does it matter so much for battery operation? In this post, we’ll dive into open-circuit potentials—a fundamental concept in battery science that underpins the voltage of a battery and how it operates.
To start, let’s revisit some subtleties in the terminology, particularly as used in Ionworks and PyBaMM, and distinguish between open-circuit potentials and open-circuit voltage.
Open-circuit voltage (OCV) of a battery: This quantity is defined for the entire battery as the electrical potential difference between the two electrodes when no current flows between them. This concept is not unique to batteries and is widely used in other electrochemical systems and electronic devices.
Open-circuit potential (OCP) of an electrode: This quantity is defined for each electrode independently. It corresponds to the OCV we would measure between the given electrode and a lithium metal counter electrode. In fact, the OCV of the battery is simply the difference between the OCPs of the positive and negative electrodes.
It’s important to note that the OCPs of each electrode (and thus the OCV of the battery) are not constant: they change as each electrode lithiates and delithiates. Higher lithiation states result in lower OCPs, while lower lithiation states correspond to higher OCPs. This is why the OCV of a battery is often used as a proxy for its state of charge (SOC), which quantifies how much energy remains in the battery. As the battery discharges, its OCV decreases.
The OCPs also play a critical role in the intercalation reactions at the surface of the active material. In simple terms, when the electrode is at its open-circuit potential, the forward and backward intercalation reactions are in equilibrium, meaning there is no net current at the interface. If the electrode potential rises above the OCP, lithium deintercalates from the particles. Conversely, if the electrode potential falls below the OCP, lithium intercalates.
This ties back to the definition of positive and negative electrodes. When a battery is discharging, the voltage drops below the battery's OCV. This means that the potential of the positive electrode is lower than its OCP, and the potential of the negative electrode is higher than its OCP. As a result, lithium deintercalates from the negative electrode and intercalates into the positive electrode.
There are, of course, many nuances we’ve glossed over here, such as the contributions of the electrolyte or the connection between OCPs and electrochemical potentials. But these are stories for another day.
When analyzing the voltage response of a battery, the OCPs of the electrodes are by far the most important components. Therefore it should not come as a surprise that they are key parameters in physics-based models of batteries. The remainder of the voltage response is composed of transient effects, including Ohmic losses, polarization, and reaction overpotentials. In fact, the kinetics of these reactions are another fundamental component of battery models—and will be the topic of our next blog post. Stay tuned!
We discussed in our previous post how the electrode with a higher potential becomes the positive electrode in a battery. But what exactly do we mean by "potential"? And why does it matter so much for battery operation? In this post, we’ll dive into open-circuit potentials—a fundamental concept in battery science that underpins the voltage of a battery and how it operates.
To start, let’s revisit some subtleties in the terminology, particularly as used in Ionworks and PyBaMM, and distinguish between open-circuit potentials and open-circuit voltage.
Open-circuit voltage (OCV) of a battery: This quantity is defined for the entire battery as the electrical potential difference between the two electrodes when no current flows between them. This concept is not unique to batteries and is widely used in other electrochemical systems and electronic devices.
Open-circuit potential (OCP) of an electrode: This quantity is defined for each electrode independently. It corresponds to the OCV we would measure between the given electrode and a lithium metal counter electrode. In fact, the OCV of the battery is simply the difference between the OCPs of the positive and negative electrodes.
It’s important to note that the OCPs of each electrode (and thus the OCV of the battery) are not constant: they change as each electrode lithiates and delithiates. Higher lithiation states result in lower OCPs, while lower lithiation states correspond to higher OCPs. This is why the OCV of a battery is often used as a proxy for its state of charge (SOC), which quantifies how much energy remains in the battery. As the battery discharges, its OCV decreases.
The OCPs also play a critical role in the intercalation reactions at the surface of the active material. In simple terms, when the electrode is at its open-circuit potential, the forward and backward intercalation reactions are in equilibrium, meaning there is no net current at the interface. If the electrode potential rises above the OCP, lithium deintercalates from the particles. Conversely, if the electrode potential falls below the OCP, lithium intercalates.
This ties back to the definition of positive and negative electrodes. When a battery is discharging, the voltage drops below the battery's OCV. This means that the potential of the positive electrode is lower than its OCP, and the potential of the negative electrode is higher than its OCP. As a result, lithium deintercalates from the negative electrode and intercalates into the positive electrode.
There are, of course, many nuances we’ve glossed over here, such as the contributions of the electrolyte or the connection between OCPs and electrochemical potentials. But these are stories for another day.
When analyzing the voltage response of a battery, the OCPs of the electrodes are by far the most important components. Therefore it should not come as a surprise that they are key parameters in physics-based models of batteries. The remainder of the voltage response is composed of transient effects, including Ohmic losses, polarization, and reaction overpotentials. In fact, the kinetics of these reactions are another fundamental component of battery models—and will be the topic of our next blog post. Stay tuned!
We discussed in our previous post how the electrode with a higher potential becomes the positive electrode in a battery. But what exactly do we mean by "potential"? And why does it matter so much for battery operation? In this post, we’ll dive into open-circuit potentials—a fundamental concept in battery science that underpins the voltage of a battery and how it operates.
To start, let’s revisit some subtleties in the terminology, particularly as used in Ionworks and PyBaMM, and distinguish between open-circuit potentials and open-circuit voltage.
Open-circuit voltage (OCV) of a battery: This quantity is defined for the entire battery as the electrical potential difference between the two electrodes when no current flows between them. This concept is not unique to batteries and is widely used in other electrochemical systems and electronic devices.
Open-circuit potential (OCP) of an electrode: This quantity is defined for each electrode independently. It corresponds to the OCV we would measure between the given electrode and a lithium metal counter electrode. In fact, the OCV of the battery is simply the difference between the OCPs of the positive and negative electrodes.
It’s important to note that the OCPs of each electrode (and thus the OCV of the battery) are not constant: they change as each electrode lithiates and delithiates. Higher lithiation states result in lower OCPs, while lower lithiation states correspond to higher OCPs. This is why the OCV of a battery is often used as a proxy for its state of charge (SOC), which quantifies how much energy remains in the battery. As the battery discharges, its OCV decreases.
The OCPs also play a critical role in the intercalation reactions at the surface of the active material. In simple terms, when the electrode is at its open-circuit potential, the forward and backward intercalation reactions are in equilibrium, meaning there is no net current at the interface. If the electrode potential rises above the OCP, lithium deintercalates from the particles. Conversely, if the electrode potential falls below the OCP, lithium intercalates.
This ties back to the definition of positive and negative electrodes. When a battery is discharging, the voltage drops below the battery's OCV. This means that the potential of the positive electrode is lower than its OCP, and the potential of the negative electrode is higher than its OCP. As a result, lithium deintercalates from the negative electrode and intercalates into the positive electrode.
There are, of course, many nuances we’ve glossed over here, such as the contributions of the electrolyte or the connection between OCPs and electrochemical potentials. But these are stories for another day.
When analyzing the voltage response of a battery, the OCPs of the electrodes are by far the most important components. Therefore it should not come as a surprise that they are key parameters in physics-based models of batteries. The remainder of the voltage response is composed of transient effects, including Ohmic losses, polarization, and reaction overpotentials. In fact, the kinetics of these reactions are another fundamental component of battery models—and will be the topic of our next blog post. Stay tuned!
We discussed in our previous post how the electrode with a higher potential becomes the positive electrode in a battery. But what exactly do we mean by "potential"? And why does it matter so much for battery operation? In this post, we’ll dive into open-circuit potentials—a fundamental concept in battery science that underpins the voltage of a battery and how it operates.
To start, let’s revisit some subtleties in the terminology, particularly as used in Ionworks and PyBaMM, and distinguish between open-circuit potentials and open-circuit voltage.
Open-circuit voltage (OCV) of a battery: This quantity is defined for the entire battery as the electrical potential difference between the two electrodes when no current flows between them. This concept is not unique to batteries and is widely used in other electrochemical systems and electronic devices.
Open-circuit potential (OCP) of an electrode: This quantity is defined for each electrode independently. It corresponds to the OCV we would measure between the given electrode and a lithium metal counter electrode. In fact, the OCV of the battery is simply the difference between the OCPs of the positive and negative electrodes.
It’s important to note that the OCPs of each electrode (and thus the OCV of the battery) are not constant: they change as each electrode lithiates and delithiates. Higher lithiation states result in lower OCPs, while lower lithiation states correspond to higher OCPs. This is why the OCV of a battery is often used as a proxy for its state of charge (SOC), which quantifies how much energy remains in the battery. As the battery discharges, its OCV decreases.
The OCPs also play a critical role in the intercalation reactions at the surface of the active material. In simple terms, when the electrode is at its open-circuit potential, the forward and backward intercalation reactions are in equilibrium, meaning there is no net current at the interface. If the electrode potential rises above the OCP, lithium deintercalates from the particles. Conversely, if the electrode potential falls below the OCP, lithium intercalates.
This ties back to the definition of positive and negative electrodes. When a battery is discharging, the voltage drops below the battery's OCV. This means that the potential of the positive electrode is lower than its OCP, and the potential of the negative electrode is higher than its OCP. As a result, lithium deintercalates from the negative electrode and intercalates into the positive electrode.
There are, of course, many nuances we’ve glossed over here, such as the contributions of the electrolyte or the connection between OCPs and electrochemical potentials. But these are stories for another day.
When analyzing the voltage response of a battery, the OCPs of the electrodes are by far the most important components. Therefore it should not come as a surprise that they are key parameters in physics-based models of batteries. The remainder of the voltage response is composed of transient effects, including Ohmic losses, polarization, and reaction overpotentials. In fact, the kinetics of these reactions are another fundamental component of battery models—and will be the topic of our next blog post. Stay tuned!
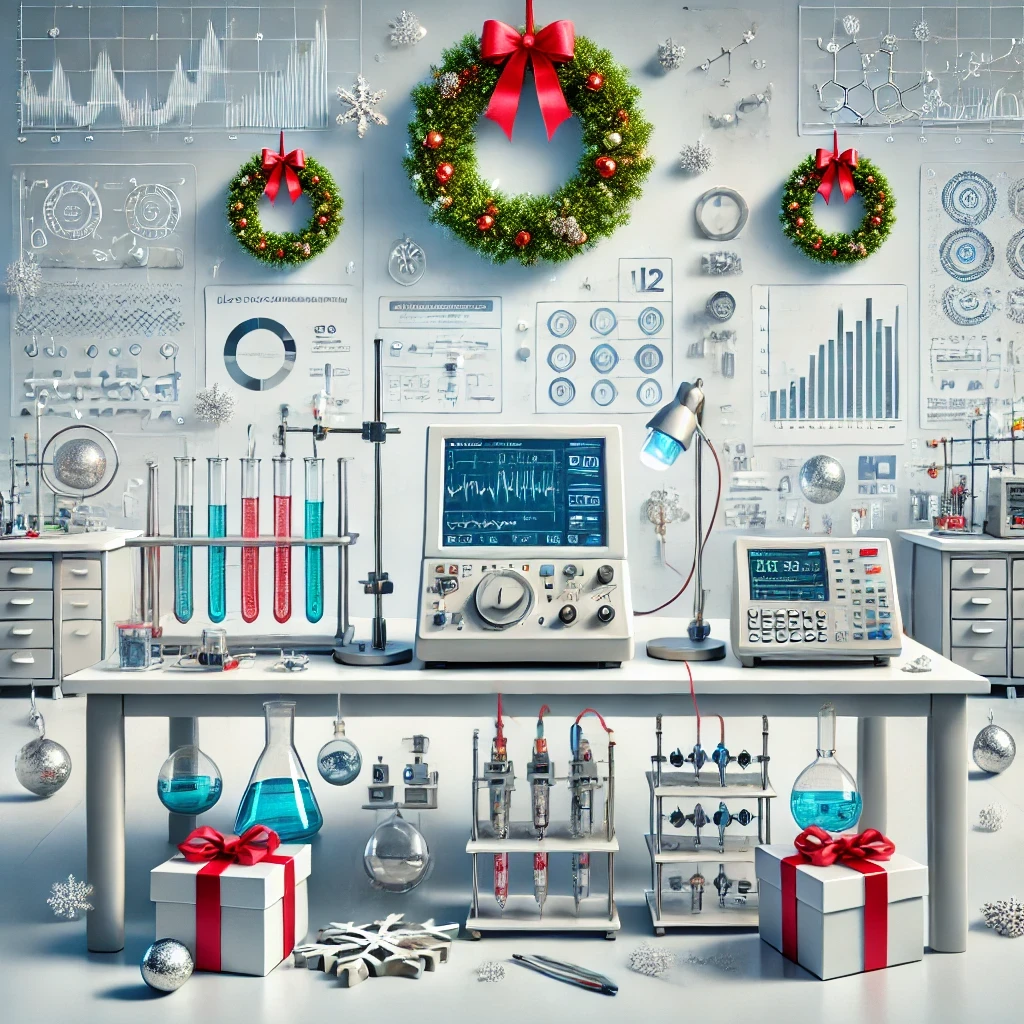
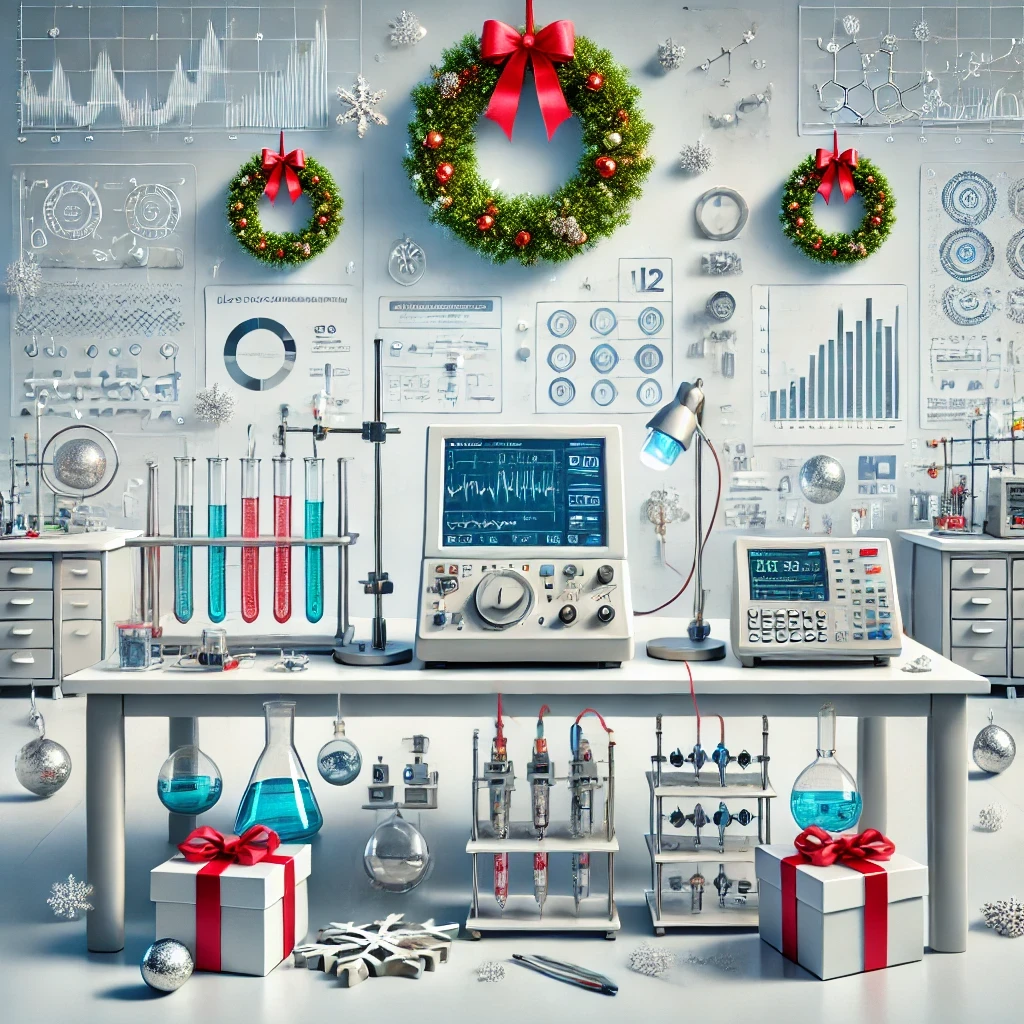
12 Days of Electrochemical Testing
To celebrate the holiday season and the re-release of Ionworks Studio, we featured "12 (business) days of electrochemical testing". Each day we pick a test, give a little bit of information about it, and show you how to run it in Ionworks. 🔋 🎄
Dec 17, 2024
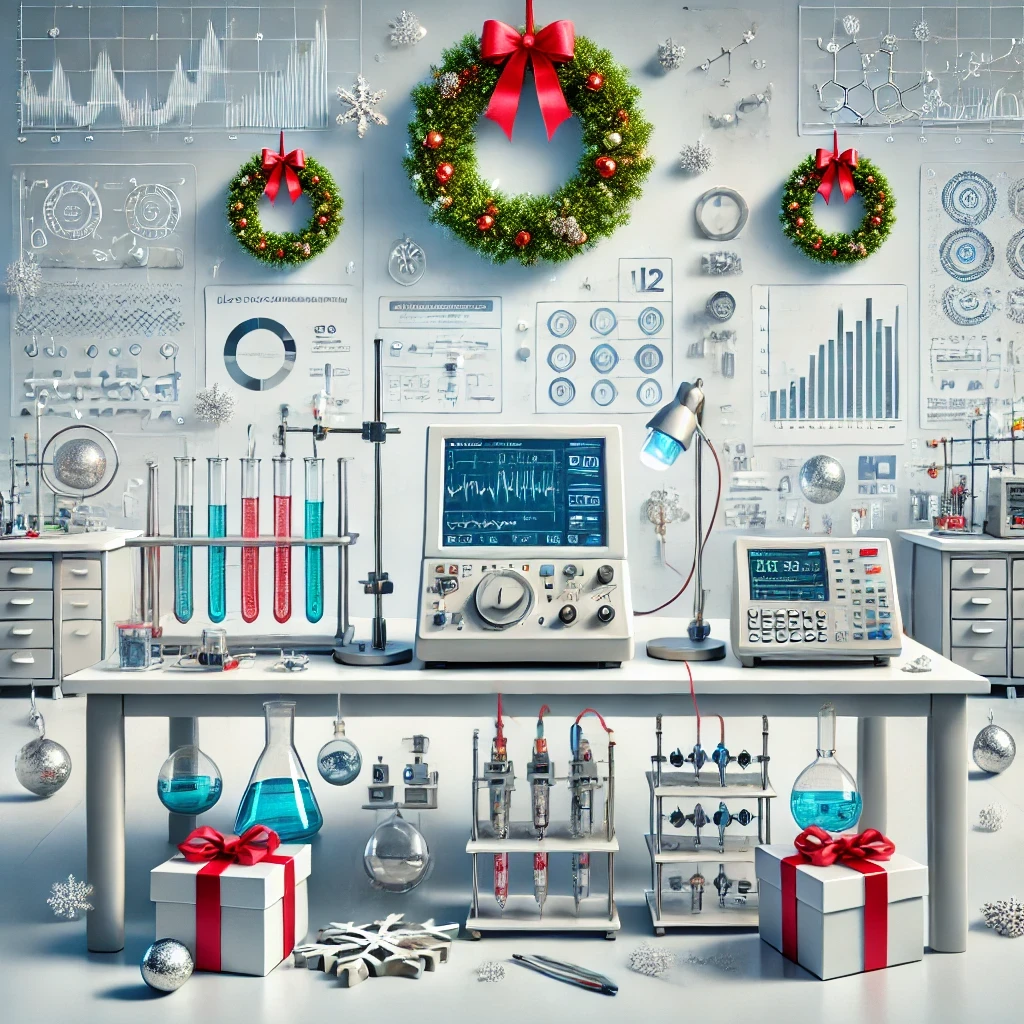
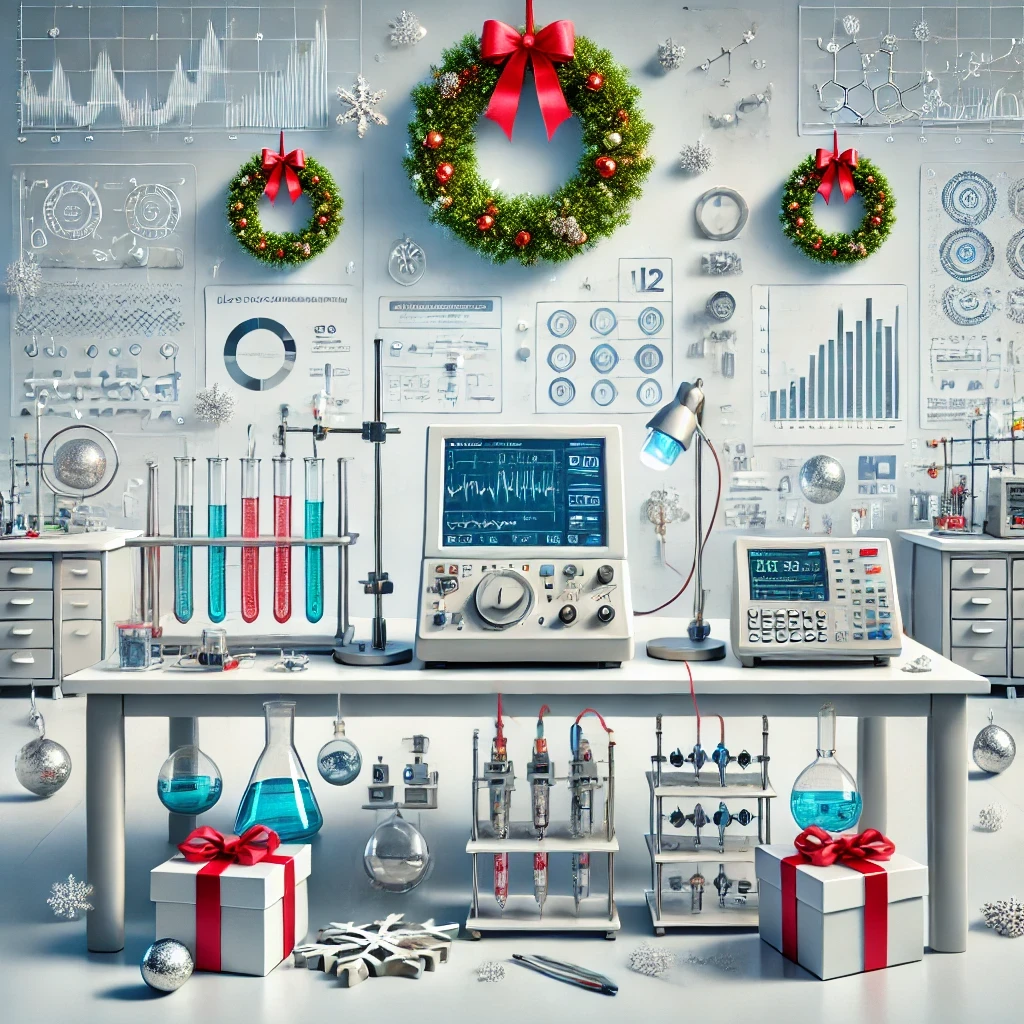
12 Days of Electrochemical Testing
To celebrate the holiday season and the re-release of Ionworks Studio, we featured "12 (business) days of electrochemical testing". Each day we pick a test, give a little bit of information about it, and show you how to run it in Ionworks. 🔋 🎄
Dec 17, 2024
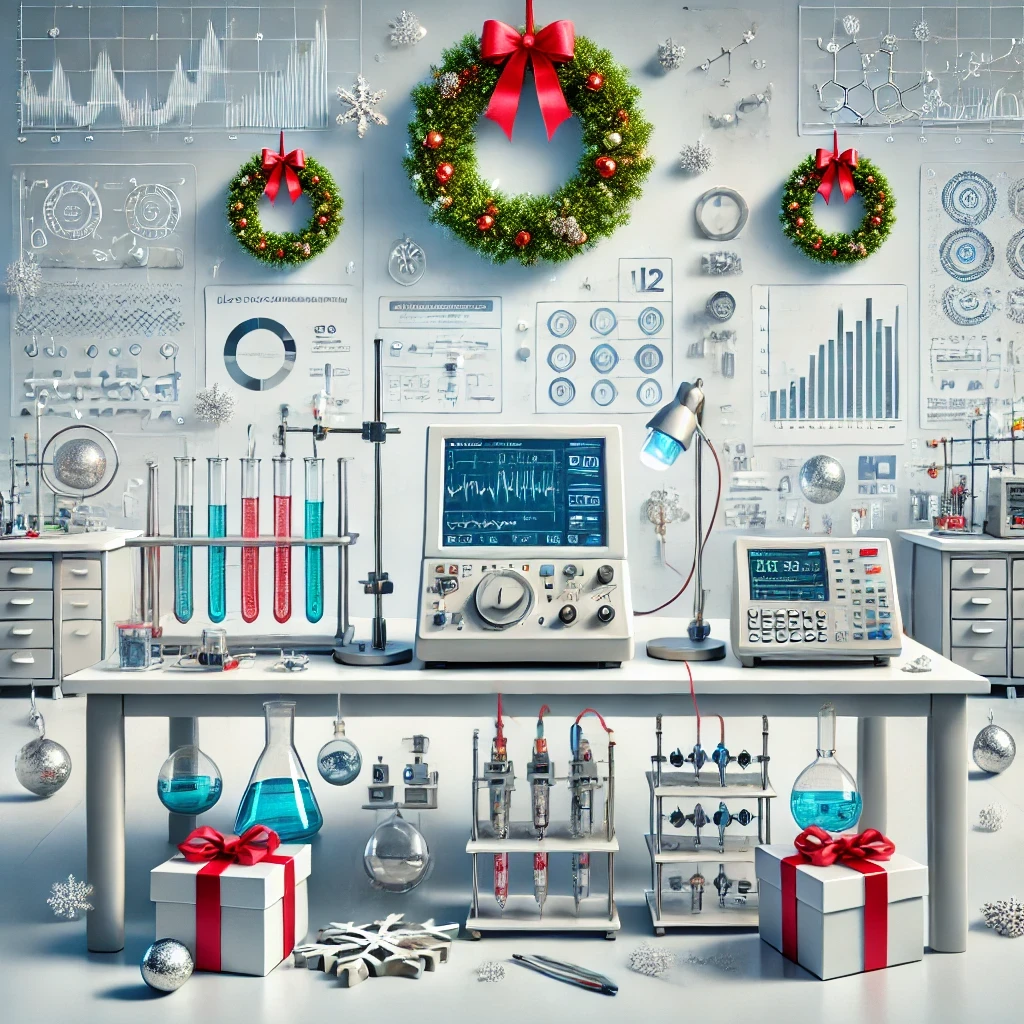
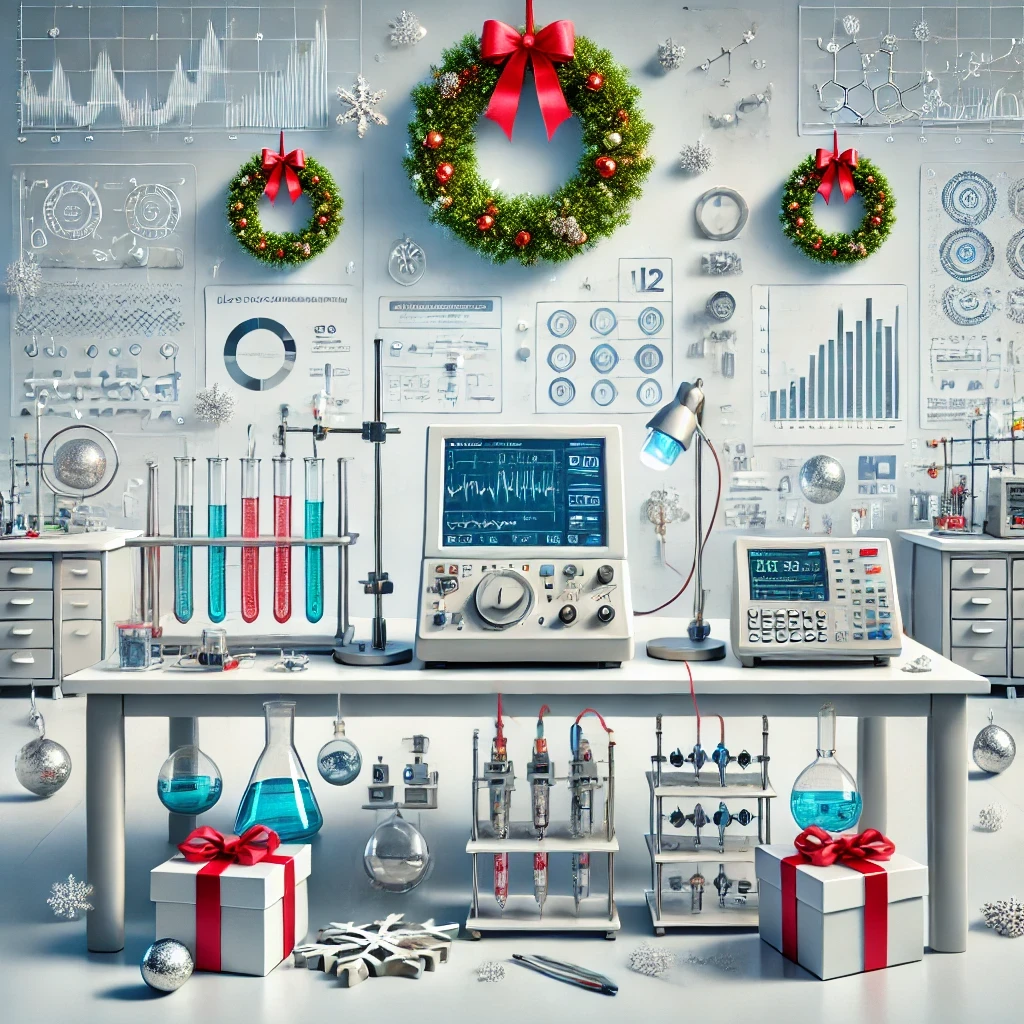
12 Days of Electrochemical Testing
To celebrate the holiday season and the re-release of Ionworks Studio, we featured "12 (business) days of electrochemical testing". Each day we pick a test, give a little bit of information about it, and show you how to run it in Ionworks. 🔋 🎄
Dec 17, 2024
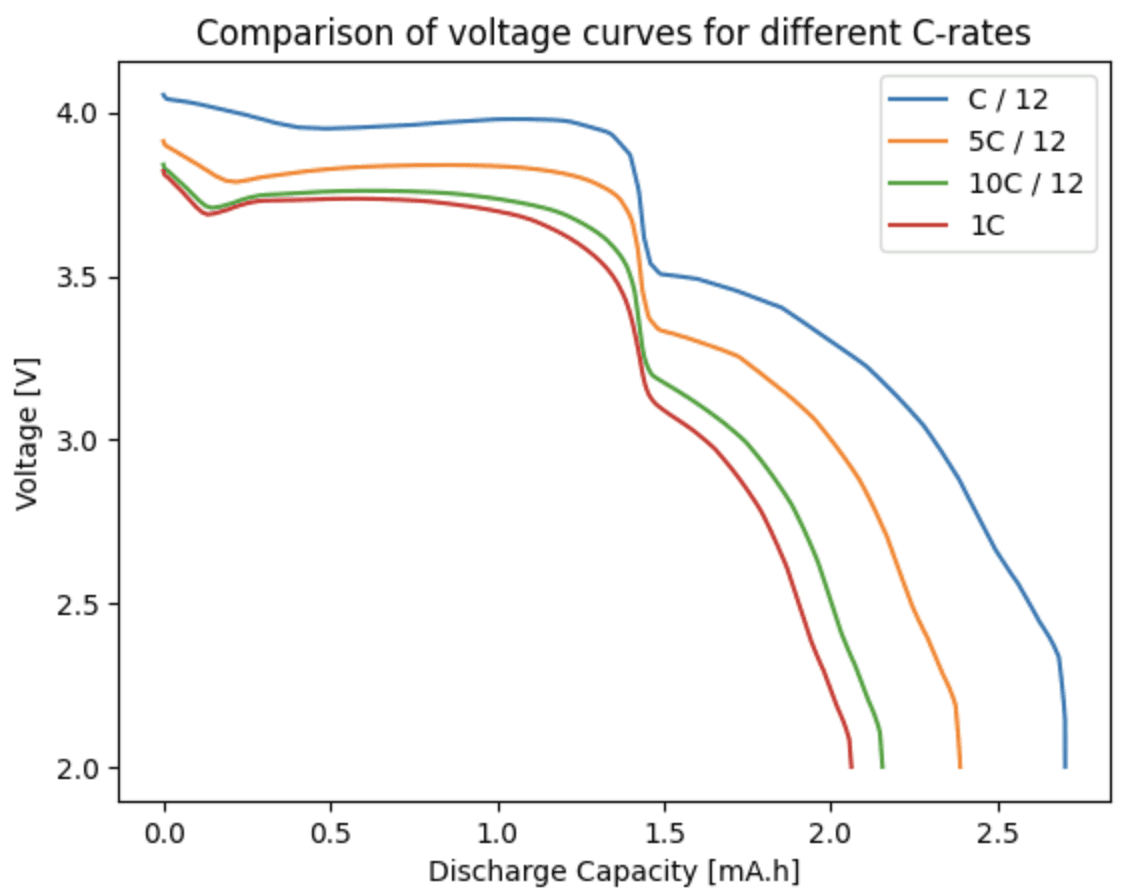
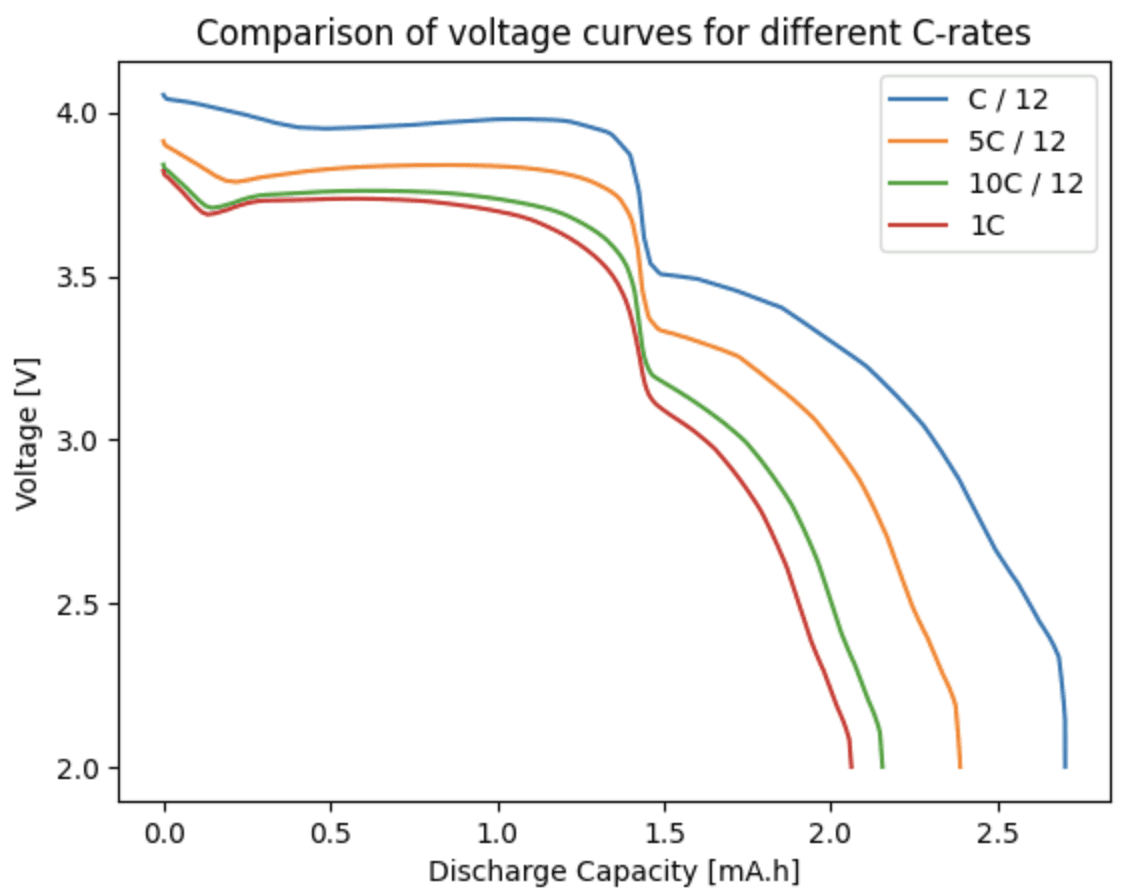
Sodium Ion Battery Model now available in PyBaMM!
This blog post explores the history of SIBs, the intricacies of the new PyBaMM model, and the exciting possibilities it unlocks for the future of energy storage.
Nov 12, 2024
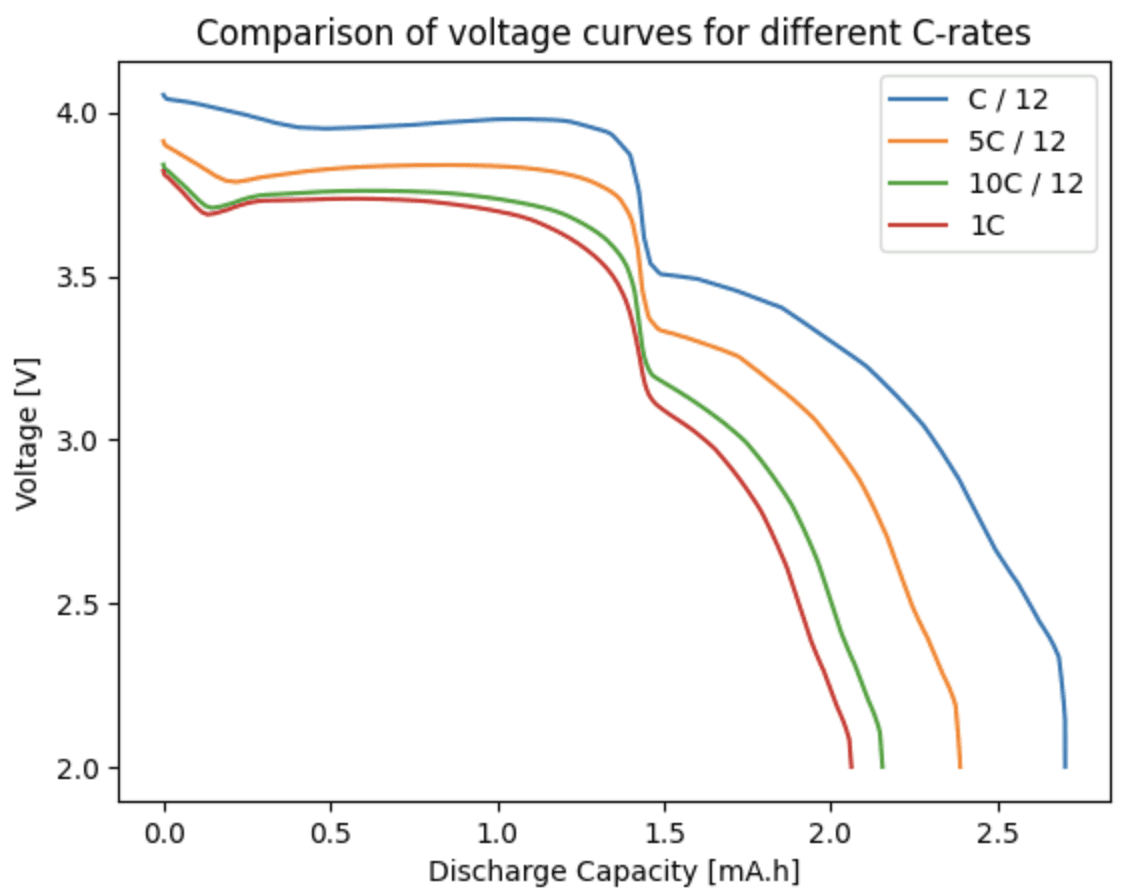
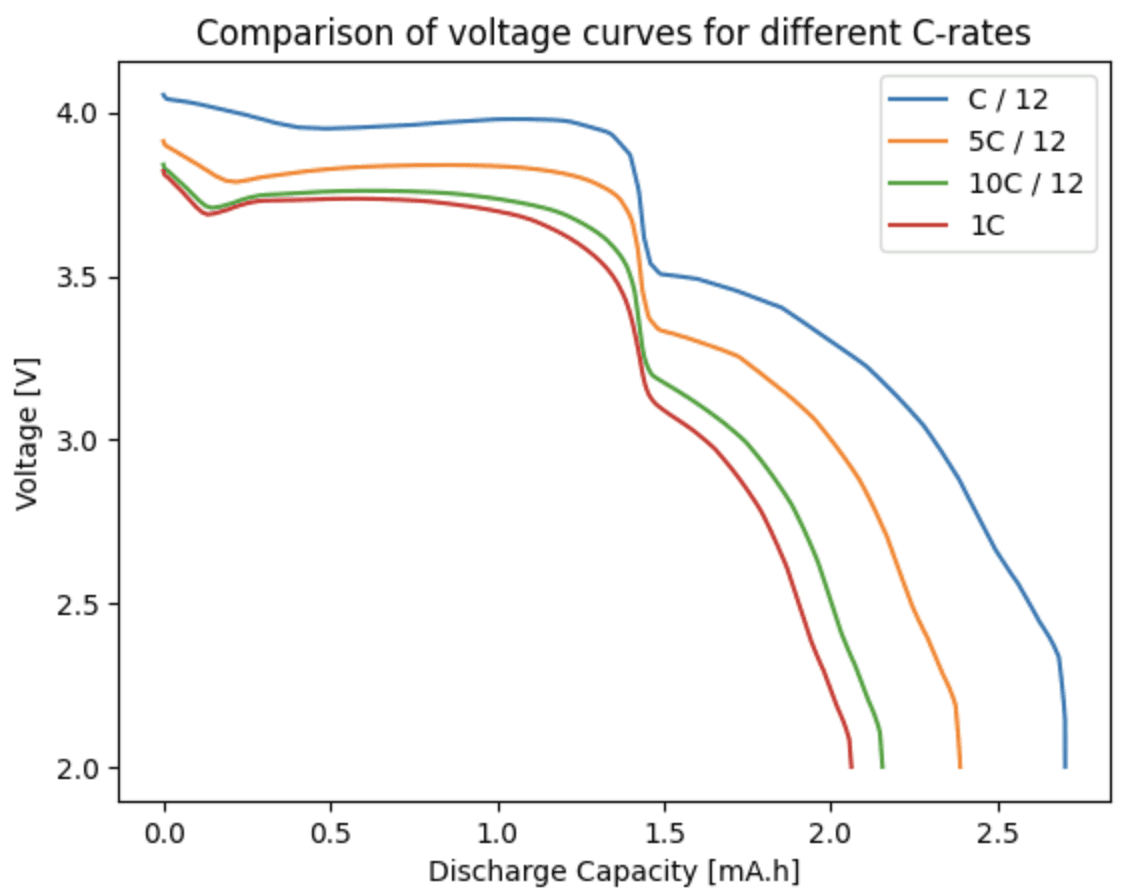
Sodium Ion Battery Model now available in PyBaMM!
This blog post explores the history of SIBs, the intricacies of the new PyBaMM model, and the exciting possibilities it unlocks for the future of energy storage.
Nov 12, 2024
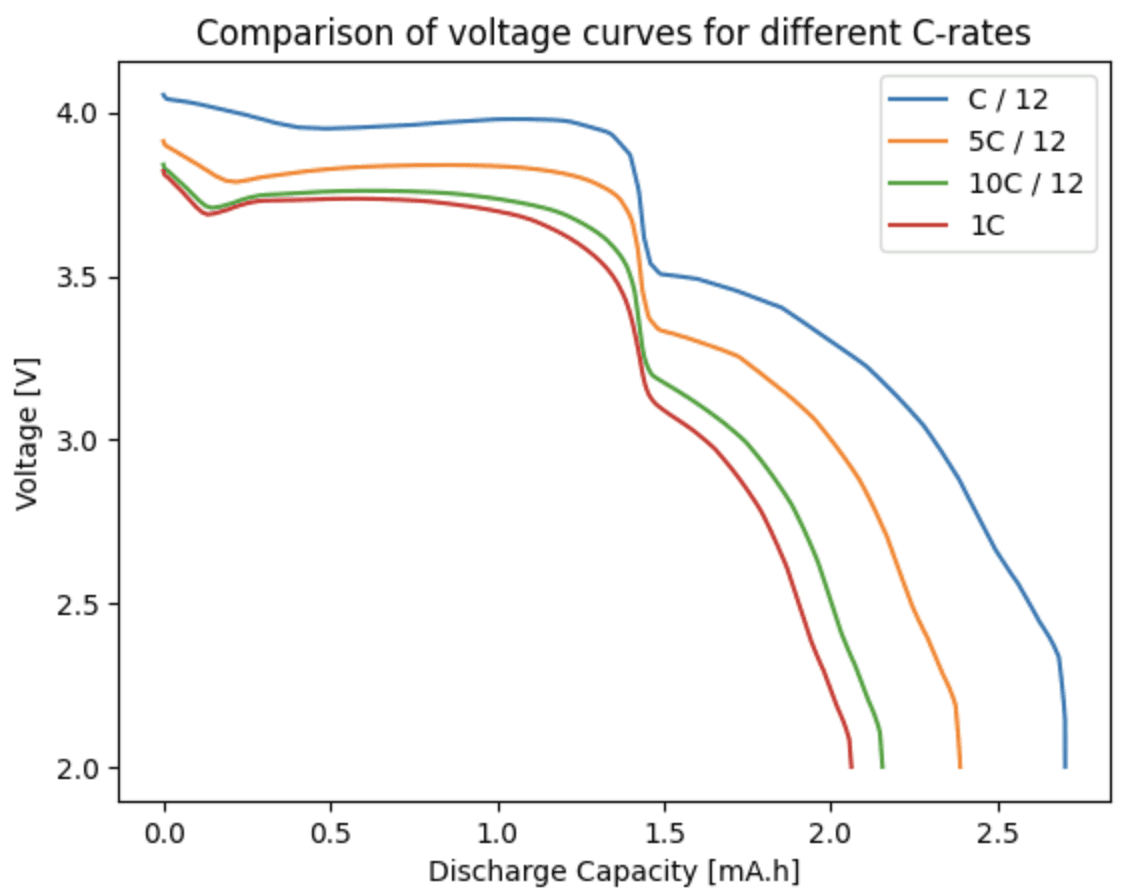
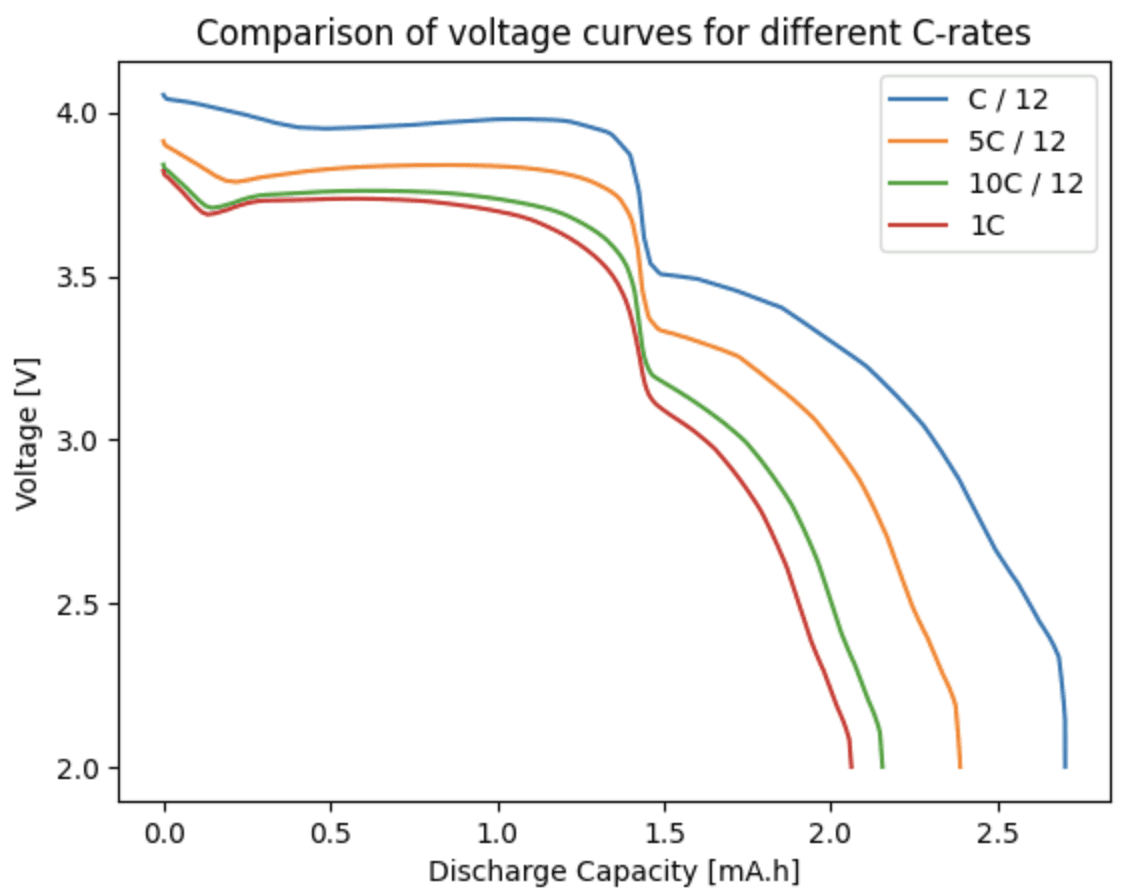
Sodium Ion Battery Model now available in PyBaMM!
This blog post explores the history of SIBs, the intricacies of the new PyBaMM model, and the exciting possibilities it unlocks for the future of energy storage.
Nov 12, 2024
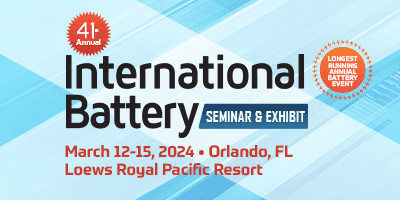
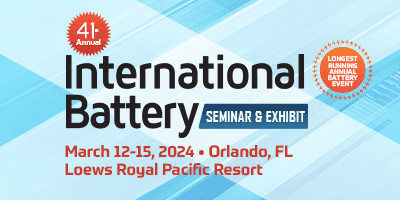
Ionworks Presents at International Battery Seminar
Ionworks CEO Valentin Sulzer presents at the International Battery Seminar in Florida
Mar 12, 2024
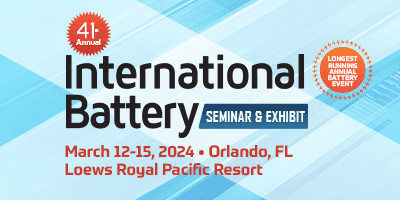
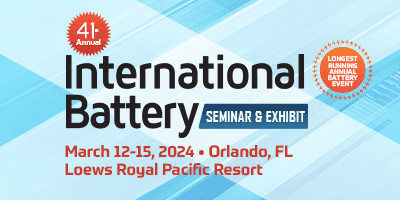
Ionworks Presents at International Battery Seminar
Ionworks CEO Valentin Sulzer presents at the International Battery Seminar in Florida
Mar 12, 2024
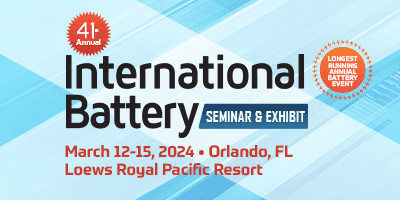
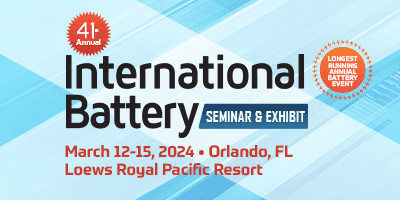
Ionworks Presents at International Battery Seminar
Ionworks CEO Valentin Sulzer presents at the International Battery Seminar in Florida
Mar 12, 2024
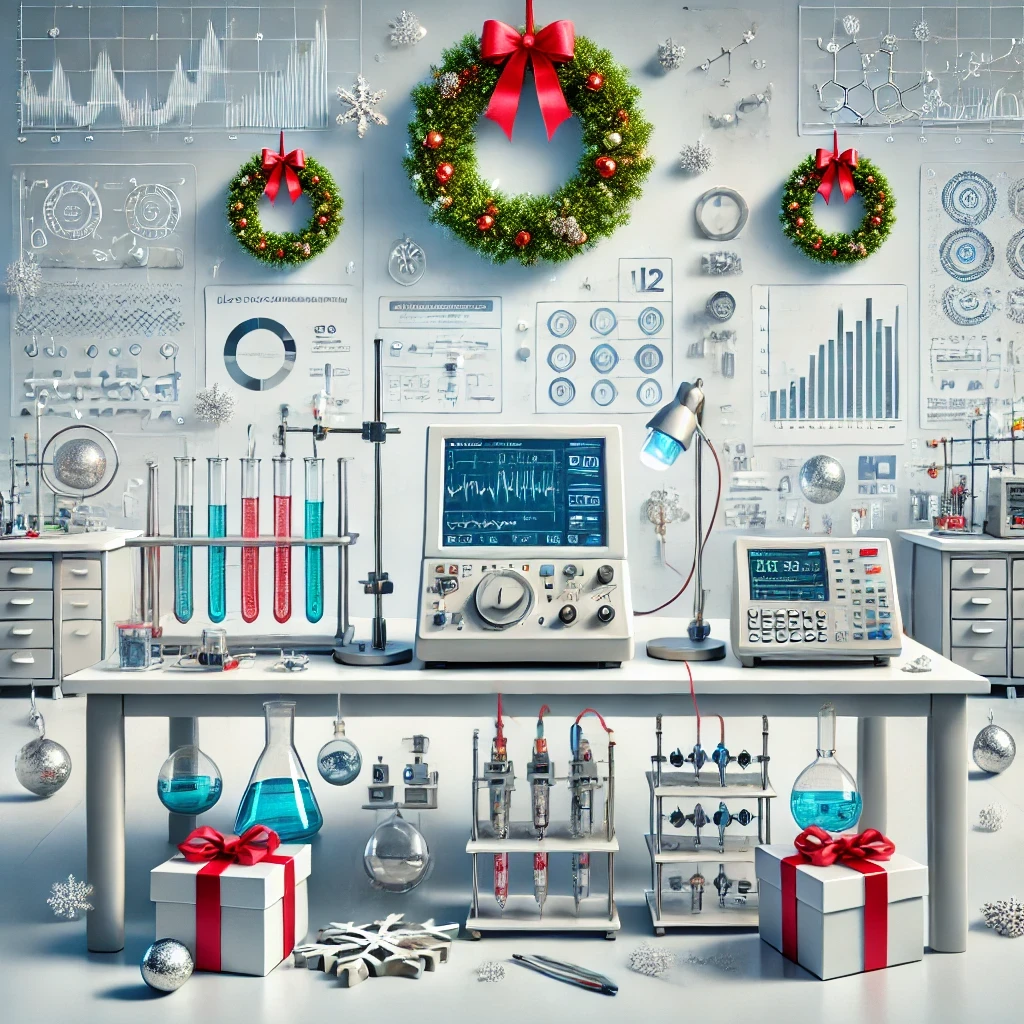
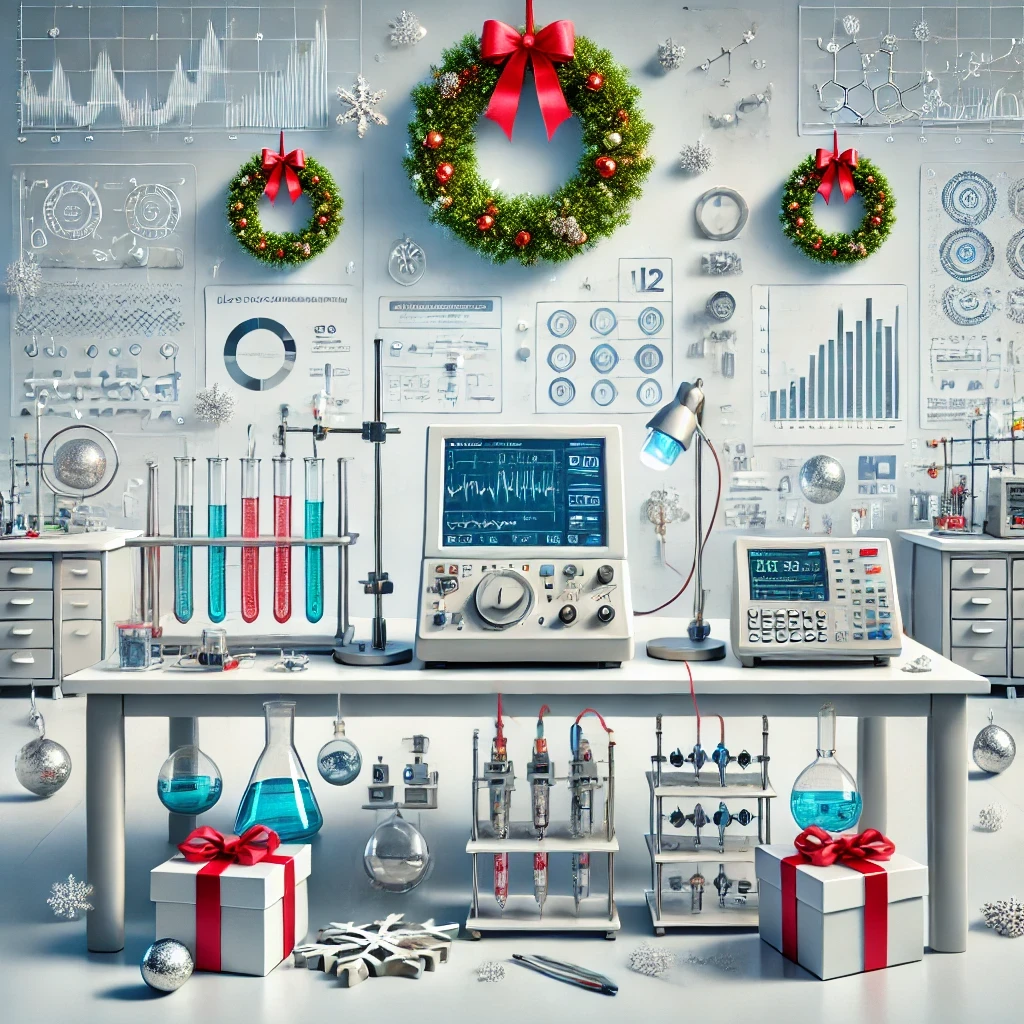
12 Days of Electrochemical Testing
To celebrate the holiday season and the re-release of Ionworks Studio, we featured "12 (business) days of electrochemical testing". Each day we pick a test, give a little bit of information about it, and show you how to run it in Ionworks. 🔋 🎄
Dec 17, 2024
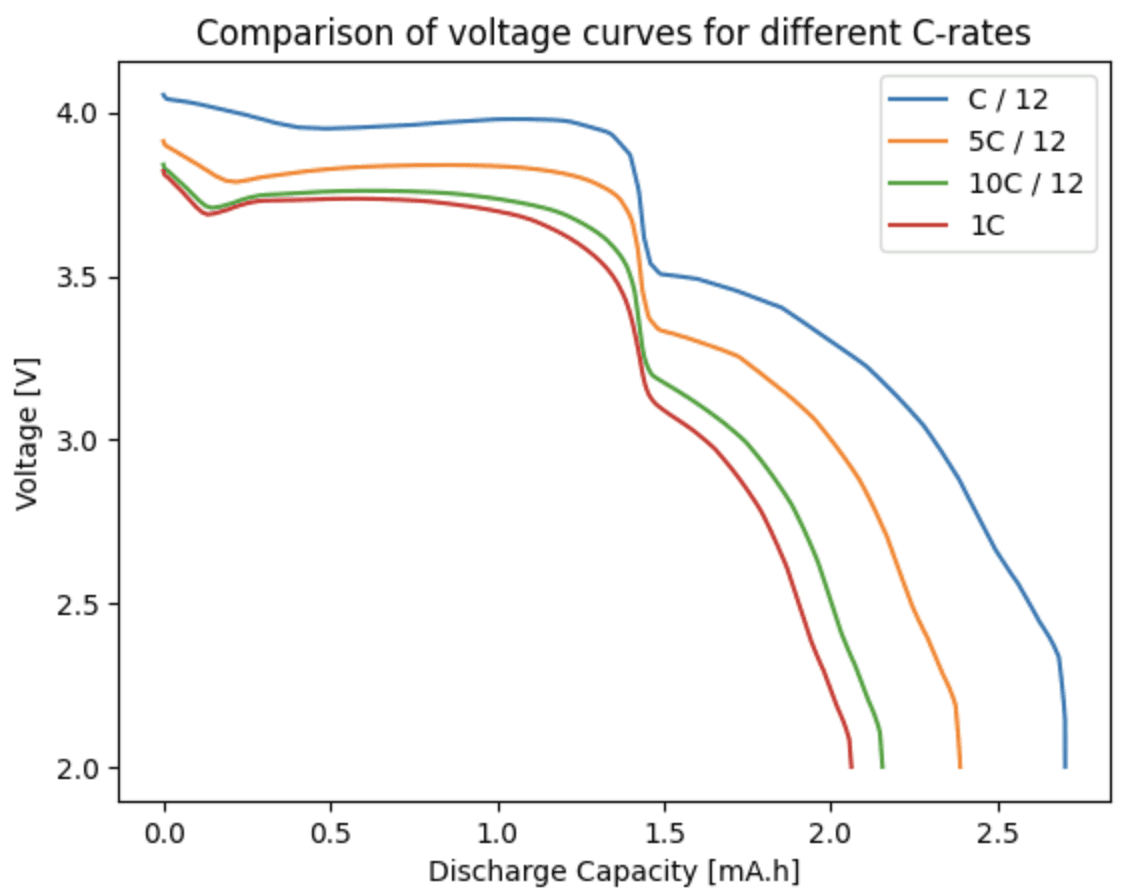
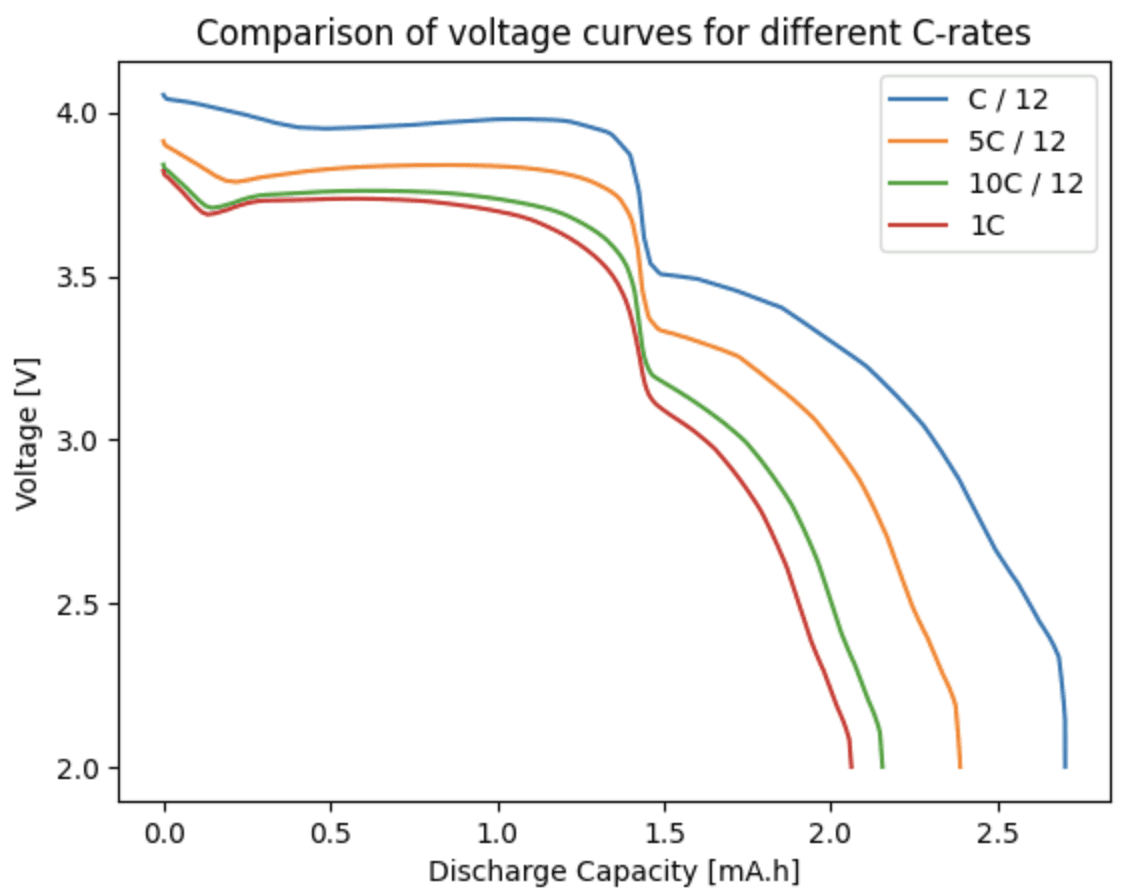
Sodium Ion Battery Model now available in PyBaMM!
This blog post explores the history of SIBs, the intricacies of the new PyBaMM model, and the exciting possibilities it unlocks for the future of energy storage.
Nov 12, 2024
Run your first virtual battery test today
Simulate, iterate, and validate your cell configurations with no lab time required.
Ionworks Technologies Inc. All rights reserved.
Run your first virtual battery test today
Simulate, iterate, and validate your cell configurations with no lab time required.
Run your first virtual battery test today
Simulate, iterate, and validate your cell configurations with no lab time required.
Ionworks Technologies Inc. All rights reserved.
Run your first virtual battery test today
Simulate, iterate, and validate your cell configurations with no lab time required.
Ionworks Technologies Inc. All rights reserved.